X-ray Imaging: Computed Tomography
Zhihua Qi, PhD
LEARNING OBJECTIVES
1. Define computed tomography (CT) number and name two materials whose CT numbers stay constant regardless of the X-ray beam energy spectrum.
2. Explain the role of bow-tie filters on a CT scanner.
3. Explain the importance of the slip ring on diagnostic CT scanners.
4. Compare analytic reconstruction with iterative reconstruction and state their differences.
5. Explain the benefits of helical scan compared to axial scan and define helical pitch.
6. Explain how tube current modulation (TCM) works and why it is desired on diagnostic CT scanners.
7. Explain how various technical parameters affect CT image quality.
8. Recognize common artifacts on CT images and understand their causes.
9. Explain the concepts of Computed Tomography Dose Index (CTDI), dose length product (DLP), and effective dose.
10. Name approaches one may take in pediatric CT to minimize unnecessary radiation.
INTRODUCTION
CT produces cross-sectional images of the object of interest through acquisition of multiple views, typically with X-ray radiation (although its principles have been widely used in applications outside of X-rays). The exploration of clinical use of CT began in the 1960s; G.T. Hounsfield and McCormack made independent investigations at that time, and were later awarded the Nobel Prize in Physiology and Medicine for their pioneering work. Since then, CT has gone through tremendous development and growth to become a highly valuable imaging tool in routine clinical use.
A number of different configurations have been used by CT; the most commonly used configuration in today’s CT scanners is referred to as the third generation (Figure 5.1). This design is also referred to as a “rotate-rotate” geometry. It is typically featured by the use of one or two assemblies of X-ray tube and detector which are fixed relative to each other and rotate around the patient for data acquisition. The X-ray beam emanating from the X-ray tube is divergent in all dimensions; usually, its divergence is discussed separately in the plane of rotation (the transverse plane or the x–y plane) and in the axial direction (or the z-direction) (Figures 5.2 and 5.3). The angle of the X-ray beam in the plane of rotation is referred to as the fan angle, usually about 60° to cover the body cross-section of a normal-sized patient. The angle of the X-ray beam in the axial direction, referred to as the cone angle, is much smaller than the fan angle. If the cone angle is so small that the X-ray beam divergence in the axial direction can be neglected without significant impact on image quality, it is often called fan beam; otherwise it is often called cone beam.
Compared to projectional X-ray imaging, CT eliminates tissue overlapping and allows an object to be characterized at each individual point, resulting in substantially improved contrast. In a reconstructed CT image, the value associated with each individual point is essentially its attenuation coefficient at the effective
X-ray beam energy used for acquisition. CT number is a quantity defined for the convenience of clinical use on the basis of X-ray attenuation coefficient. Its definition is
X-ray beam energy used for acquisition. CT number is a quantity defined for the convenience of clinical use on the basis of X-ray attenuation coefficient. Its definition is
![]() FIG. 5.1 • The illustration of the third-generation computed tomography. Reprinted with permission from Orth D. Essentials of Radiologic Science. 2nd ed. Philadelphia, PA: Wolters Kluwer; 2017. |

where µ and µwater are the X-ray attenuation coefficients of the image point and water, respectively, at the effective X-ray beam energy used for acquisition. By definition, CT numbers for water and air are constant regardless of X-ray beam energy. For water, its CT number is always zero, whereas for air, its CT number is always −1000. Other than these two materials, CT number for a certain material generally varies by X-ray beam energy. Typical CT numbers of human tissues are shown in Figure 5.4.
CT SYSTEM
The components of a modern CT system include the X-ray tube, the detector, the gantry, the data acquisition system (DAS), the patient table, the control console, the reconstruction engine, and others. The inside of a CT scanner when the cover is off is shown in Figures 5.5 and 5.6. Several of these components are discussed in more detail later.
X-ray tube
As the X-ray-producing component of the system, the X-ray tube produces sufficient flux of X-ray photons at desired energy levels within a typically short scan time. It is important for the X-ray tube to be both powerful and robust to meet the clinical needs in a radiology department. The performance of the X-ray tube affects both image quality of the scans and radiation dose received by patients. Discussions of several important aspects regarding the tube are provided as follows:
Heat management—the majority of the input electrical energy is converted into heat in the process of X-ray production. An X-ray tube of high performance should be able to withstand high temperature and dissipate heat rapidly and efficiently. The use of liquid metal bearings is one example of X-ray tube designs that promote heat management. Liquid metal bearings support the anode and facilitate its rotation during an exposure, as conventional ball bearings do, but offer much improved efficiency in the conduction of the produced heat.
X-ray beam energy—the energy spectrum of X-ray photons produced by an X-ray tube is mostly continuous within its range, with several discrete peaks characteristic of the anode material (Figure 5.7). The tube voltage, applied between the anode and the cathode, defines the maximum energy of the spectrum. The mean energy of the X-ray beam, in the unit of keV, is approximately between 1/3 and 1/2 of the kVp value. Most often, 120 kVp is used for head and body examinations of typical adult patients, but other kVps are available for the consideration of various patient sizes and different clinical tasks. On clinical scanners, 80 to 140 kVps in the increment of 20 kVp are typical offerings, but 70 and 150 kVp have become available on some newer models for advanced needs.
Focal spots—two filaments are usually available in the X-ray tube to provide dual focal spots for imaging. The small focal spot is used for applications that require high spatial resolution, like imaging of extremities and small bony structures, whereas the large one is used for those that require high flux of X-ray photons, like body imaging. During the flight of high-speed electrons from the cathode to the anode, the electron beam may have broader lateral spread by the time it reaches the anode because of the repulsive force between electrons. Both kVp and mA affect the lateral spread, so the focal spot varies for different combinations of kVp and mA settings. Newer technologies have been introduced to allow active control of the lateral spread and therefore more constant focal spot size regardless of the choices of kVp and mA.
X-ray beam collimator
Collimators are important components of a CT system. A CT scanner has both a prepatient collimator and a postpatient collimator, which work together to limit radiation to only the area of clinical interest (Figure 5.8). The use of a postpatient collimator is necessitated because the focal spot usually has a finite size and produces nonnegligible penumbra beyond the boundaries defined by the prepatient collimator.
X-ray collimation is also served by the antiscatter grid, mounted on the surface of the detector. The purpose of an antiscatter grid is to keep scattered photons from reaching the detector for image quality improvement. 1D grids are common, although some high-end models have employed 2D grids for improved reduction in scatter.
X-ray beam filter
Filters are devices mounted at the beam exit port of an X-ray tube to further modify the intensity and energy characteristics of the X-ray beam before it interacts with patients.
Two types of filters are typically used. The first is a thin flat filter made of metal and applied to “harden” the X-ray beam by preferentially removing low-energy photons in the beam. The second is often called a bow-tie filter, whose profile is to account for the oval shape of the human body. When a homogeneous X-ray field is incident upon the human body, the center of the detector receives the lowest signal because of the longest X-ray attenuation path, whereas peripheral areas of the detector corresponding to body boundaries receive the highest signal because of little attenuation along the path. This is undesired because the range of signals received by the detector may be too wide to handle and the body periphery receives much higher radiation than is necessary. The bow-tie filter provides an effective solution to this problem by equalizing the X-ray flux across the detector within the axial plane (Figure 5.9). A CT scanner typically has several bow-tie filters optimized for different body sizes.
Detector
Solid-state detectors are the most common type of detectors used on diagnostic scanners today. These detectors can use either an indirect conversion or direct conversion process for X-ray detection (Figure 5.10).
Indirect conversion detector
Indirect conversion detectors are used in nearly all clinical CT scanners today. With this technology, a detector is made of a block of scintillating material coupled to a photodiode array. An incident X-ray photon undergoes a photoelectric interaction with the scintillator, and, as a result, produces a freely moving photoelectron, which goes on to excite electrons in other atoms. When these excited electrons return to their ground state, characteristic photons are emitted in the visible or ultraviolet light spectrum. Those light photons traveling toward the photodiode array will
convert their energies to electrical signals, which are subsequently processed, digitized, and stored by the electronics of the DAS.
convert their energies to electrical signals, which are subsequently processed, digitized, and stored by the electronics of the DAS.
The light photons produced by the scintillation process travel in all directions. To direct more light photons toward the photodiode array, the scintillator block is often divided into an array of columns and reflective coatings are applied at the boundaries of individual columns. When light photons travel in directions other than the forward, they will be reflected toward the photodiode array for higher detection efficiency.
Geometric configuration
A CT detector is a 2D array of individual detector elements or pixels. It can be viewed as multiple identical rows stacking up along the longitudinal axis (z-axis). Scanners nowadays typically have 16, 32, 64, 128, or more row of detectors, and within each row, there are thousands of detector pixels distributed along an arc (˜60°) concentric to the X-ray source (if the source is at the same plane defined by the arc). The detector pixel, the smallest unit of X-ray detection, has comparable sizes in both dimensions; when projected to the isocenter, the pixel sizes are typically within the range of 0.5 to 0.625 mm.
The configuration of detector rows in the longitudinal direction varies by both hardware design and clinical use. This is illustrated in Figure 5.11.
Detector performance
The performance of the detector closely impacts image quality of CT. Some clinically relevant aspects of its performance are discussed as follows:
Primary speed—during the acquisition of each projection view, the scintillation process takes time to complete before the scintillator can return to its ground state and be ready for the acquisition of the next view. In general, the faster the primary speed of a detector is, the less contamination from previous views
there is in the data for each view. For those studies that require the fastest rotation speed and/or the highest view sampling rate a scanner can afford, detector primary speed needs to be fast enough to reduce or eliminate blurring caused by signal contamination between neighboring views.
Radiation history—the gain of a detector changes after its scintillator is exposed to X-ray flux. Although such changes may be subtle within a short period, they can result in substantial differences across the detector array and therefore in artifacts on CT images. This issue can be addressed by (1) avoiding unnecessary exposures to detector channels by choosing the appropriate scan field of view and (2) routine calibrations or corrections. New materials insensitive to radiation damage are also of interest to minimize radiation history-related artifacts.
Uniformity—the pixels of a detector are typically grouped into channels along the angular direction. Channel-to-channel uniformity is crucial in producing artifact-free CT images. If the response of a channel to radiation deviates substantially from that of the others, artifacts arise on images, typically in the form of arcs or rings on the images.
Cross-talk—ideally, the signal of a detector pixel should be separate and independent from that of its neighboring pixels, but, in practice, there are often leakage signals for each pixel from its neighbors. Cross-talk causes loss of spatial resolution and degradation of image quality.
Direct conversion detector
In recent years, direct conversion detectors have been extensively investigated for their use in CT. This type of detector is made of a layer of photoconductor, like selenium and cadmium zinc telluride, with a bias voltage applied across the detector structure. The X-ray photons generate electron-hole pairs in the photoconductor layer and each pair, once generated, is swiftly swept to the electrodes by the electric field to produce its electrical signal. When built with ultrafast readout electronics, the direct conversion detector may allow each individual photon to be measured for its energy, which opens up opportunities for applications like multienergy imaging, low-dose CT, and so on. One of the major challenges faced by this excitingly new technology includes the robustness of the detector and its capability of handling high X-ray photon flux.
Gantry
The gantry of a modern CT scanner needs to support high-speed transfer of power, data, and signals. At any time during a CT scan, between the moving parts, rotating at high speed, and the stationary gantry, large amounts of electrical energy, data, and control signals need to be transferred within a very short time. Slip ring is an enabling technology for high-speed continuous rotation on a CT scanner (Figure 5.12). Slip ring includes essentially high-performance electrical brushes that maintain close contact between the moving and nonmoving parts and ensure timely transmission between them.
The gantry also requires high mechanical standards to fulfill the requirements of clinical CT scans. The rotating components of a CT scanner can easily weigh over 1000 lb, and, while such a large load is rotating at high speed, high degrees of mechanical accuracy and precision are also desired. A simple fact in the mechanical design is that the centrifugal force a gantry needs to withstand increases with the square of the rotation speed. Therefore, for a scanner to improve its rotation time from 0.35 to 0.25 seconds, that is, an improvement by 40%, the centrifugal force the gantry has to withstand nearly doubles.
CT RECONSTRUCTION
There are largely two types of reconstruction algorithms for CT, analytic and iterative reconstruction algorithms. Filtered back projection (FBP) is the basis of most analytic algorithms and has been used as primary for a long time on clinical scanners. Iterative algorithms did not gain popularity in early days of CT, but have moved rapidly into clinical practice in recent years, mainly due to advances in computation speed and demands in imaging with lower radiation dose.
Analytic reconstruction
Back projection
Back projection (BP) is not practically used, but, as an intuition-based concept, is helpful to the understanding of the later introduced FBP. With this algorithm, the reconstructed image starts as a matrix of zeroes at all locations. Then, at each view angle and for each detector element, the image pixels encompassed by the X-ray beam illuminating the detector element are identified, and the value of the detector element is added to each of these identified pixels. By projecting the signal back along its path, the process is intuitively a reversal of the signal production. One may easily notice that BP reconstructs a blurred version of the original image (Figure 5.13). Such a blur comes as no surprise in that, along each ray path, each image pixel contributes differently to the value of the detector pixel, and such nonuniformity is lost while the same value is back projected to each image pixel in the reconstruction. A deblurring step is necessary to ensure the exactness of reconstruction.
Filtered back projection
FBP is the basis of the vast majority of analytic algorithms used on CT scanners. An FBP algorithm consists of two steps: first, in the “filtering” step, projection data of each view, are filtered by a specially designed function, often called “filter” or “kernel,” and then, in the “back projection” step, each detector element, with its data filtered, back projects and adds its contribution to the pixels along the ray path that produces its signal. With the added filtering step, FBP is able to give a theoretically exact reconstruction of the image (Figure 5.13).
The concept of k-space, the concept used extensively by magnetic resonance imaging, is helpful in understanding how FBP works. It is a domain in which any object is viewed as a summation of spatially oscillating patterns of various frequencies and characterized by its intensity distribution over these different frequencies. Assuming a CT scan in which a parallel x-ray beam (a beam of x-rays parallel to each other) rotates around the patient, the projection data acquired at each view is essentially a line in the k-space which goes through the origin and is angled specifically to the view angle; with projection data from all different views, the k-space counterpart of the desired image can be assembled and the image recovered through a well-defined mathematical transform, aka the Fourier transform. This is called central slice theorem, as is illustrated in Figure 5.14. In short, the problem of reconstructing an image turns into solving its k-space counterpart followed by a well-defined transform back into the spatial domain. In practice, one could only acquire a finite number of view angles, and, at each of them, acquire data at only a finite number of points. With the view angles and data points on each view typically evenly spaced,
k-space regions near the origin (low-frequency regions) are covered at a density higher than those far away (high-frequency regions). To compensate for this nonuniformity in sampling density, it becomes necessary to give higher weighting to high-frequency data points than to low-frequency ones at each view angle. This explains why filtering is a necessary step of the reconstruction and why the applied filter is in a “ramp” shape, namely, it increases linearly, from zero at the origin toward higher frequency.
k-space regions near the origin (low-frequency regions) are covered at a density higher than those far away (high-frequency regions). To compensate for this nonuniformity in sampling density, it becomes necessary to give higher weighting to high-frequency data points than to low-frequency ones at each view angle. This explains why filtering is a necessary step of the reconstruction and why the applied filter is in a “ramp” shape, namely, it increases linearly, from zero at the origin toward higher frequency.
![]() FIG. 5.14 • The central slice theorem. FT, Fourier transform. Reprinted with permission from von Schulthess GK. Molecular Anatomic Imaging. 3rd ed. Philadelphia, PA: Wolters Kluwer; 2016. |
Although the filter is, in theory, a ramp function to ensure the exactness of the reconstruction, it is often modified to enhance certain aspects of image quality depending on the clinical application. A “body” filter is designed to suppress noise and give the resulting images a smoother look to resolve subtle signal differences between lesions and normal tissues in body examinations. This filter is nearly the same as the ramp filter in the low-frequency range, but as the frequency gets higher, it starts to roll off instead of continuing to increase for the desired suppression of noise in the high-frequency range. A “bone” filter, on the other hand, is designed to enhance features of tiny sizes to detect bone fractures, calcified specks, and others alike. Therefore, one should expect higher values in the high-frequency range of the filter compared to those of the ramp filter.
FBP algorithms gain popularity in their clinical use because of simplicity and speed. At the same time, their simplicity, mostly due to assumptions of rather ideal systems, has led to limitations in addressing issues affecting practical systems.
Iterative reconstruction
In an iterative algorithm, models accounting for practical aspects of a CT scan are incorporated into the reconstruction process. As an analytic solution in one step becomes unattainable, an iterative algorithm utilizes a numerical approach to solve for the desired image; this approach starts with an estimated image, and iteratively updates it following a model-driven guidance until the level of consistency between the image and the projection measurements becomes acceptable. Figure 5.15 illustrates a typical workflow of iterative reconstruction.
Compared to the standard FBP algorithms, iterative reconstruction algorithms are able to make improvements in image quality through lowering image noise, suppression of artifacts, and others. Their capability of lowering image noise can be translated to reduction in radiation dose to patients. Despite the potential clinical benefits of iterative reconstruction algorithms, special attention should be paid to their uses in clinical applications, with considerations such as (1) they may introduce substantial change in the texture of image noise, which may produce undesired artifacts and interfere with visual detection of subtle features on images; (2) they may introduce changes in the quantitative aspects of CT images and make necessary changes in the existing standards on the basis of traditional algorithms. The capability of radiation dose reduction by an iterative reconstruction algorithm needs to be carefully evaluated for each diagnostic task with considerations of their impact on diagnostic performance.
CT TECHNIQUES
Axial scan
In an axial scan (Figure 5.16), the tube-detector assembly rotates around the patient while the patient couch stays still. To cover
anatomy longer than the longitude coverage of a single axial scan, a step-and-shoot mode may be employed, in which the couch steps through a series of locations with an axial scan performed at each step but with no radiation between steps.
anatomy longer than the longitude coverage of a single axial scan, a step-and-shoot mode may be employed, in which the couch steps through a series of locations with an axial scan performed at each step but with no radiation between steps.
![]() FIG. 5.15 • A typical workflow of iterative reconstruction. Reprinted with permission from Chandra R, Rahmim A. Nuclear Medicine Physics: The Basics. 8th ed. Philadelphia, PA: Wolters Kluwer; 2018. |
Helical scan
In a helical scan (Figure 5.17), the tube-detector assembly rotates around the patient while the patient is continuously translated through the center of rotation. It allows a fast scan through an anatomic volume.
Helical pitch is a common concept used in the characterization of how fast the patient table moves relative to the width of the detector coverage. It is defined as the table feed per 360° gantry rotation divided by the width of the detector coverage at the isocenter.
Cine scan
A cine scan (Figure 5.18) refers to a series of axial scans at the same couch location for the characterization of temporal changes in the anatomy of interest.
Gated scan
With a gated scan, exposure to the patient is made only when the patient is at a desired physiologic state. A gated scan is often desired in imaging organs subject to breathing and/or cardiac motion. The differences in the images when the cardiac structures are scanned with and without gating are shown in Figure 5.19.
Although a gated scan may intuitively mean a scan in which the beam is only on at certain time points, it may refer to two different scan modes. The first is the prospectively gated scan, as the intuitive meaning of a gated scan. The other is the retrospectively gated scan, in which the beam is always on (may vary in its X-ray flux), but images for a certain physiologic state may be retrospectively reconstructed using only the data acquired at the intended state. Illustrations of the two different modes are shown in Figure 5.20.
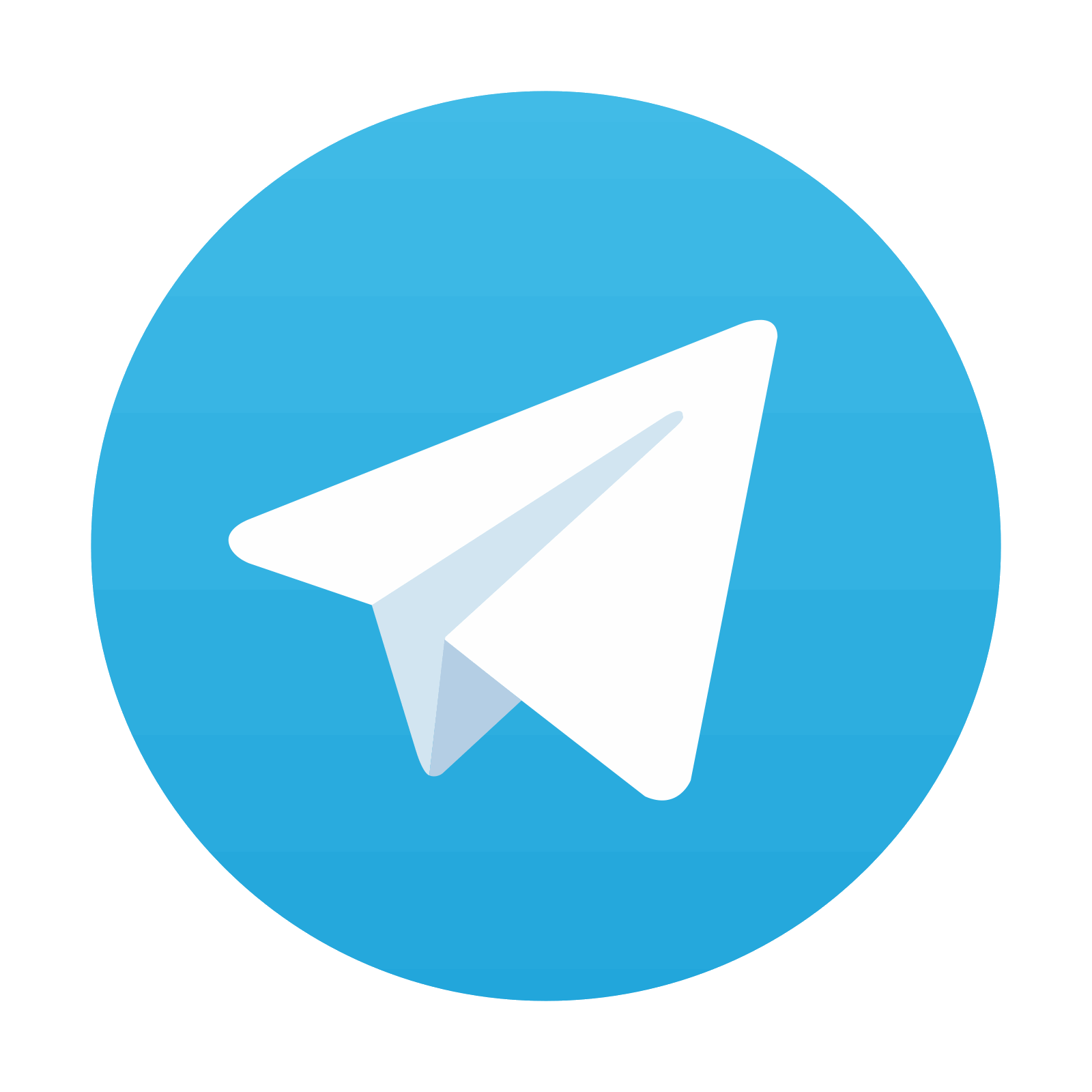
Stay updated, free articles. Join our Telegram channel
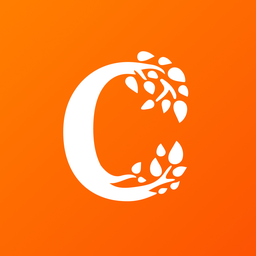
Full access? Get Clinical Tree
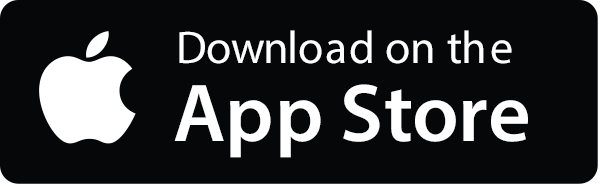
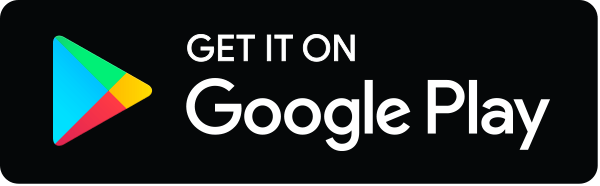