Key points
- •
The vertebral endplate is the key regulator of disc nutrient and oxygen transport.
- •
The endplate is composed of an inner bony and an outer cartilaginous layer in relation to the vertebra.
- •
Vertebral endplates are innervated so represent an important, yet often overlooked, potential source of pain.
- •
Endplate pathological change and damage may precede disc degeneration; however, endplate changes may also develop following disc changes and may also promote the development of other clinically relevant imaging phenotypes, such as Modic changes.
- •
A standardized definition of structural endplate abnormalities and defects does not exist but is very much needed to aid our understanding of their role in spine disorders, their relation with other spine phenotypes, and/or the various clinical manifestations of pain/disability as well as treatment outcomes.
The vertebral endplate is a thin layer of tissue interposed between the hard vertebral body and the soft intervertebral disc (IVD) (see Chapter 1 ) [ , ]. The healthy endplate is a composite structure containing both an inner bone and outer cartilaginous layers in relation to the vertebra [ ]. As such, the endplate is the key nutrient transport for the disc, maintains the stress–strain relationship between the disc, prevents the loss of macromolecules, facilitates the structure of the IVD, and is a key pain-generating source. Abnormalities, defects, and/or changes of the endplate can lead to IVD degeneration or be a consequence of it, but more importantly can lead to the development of pain and disability that may necessitate clinical intervention [ ]. Endplate abnormalities/defects/changes have been found to be heritable and at times may be a precursor to the development of IVD degeneration (see Chapter 6 ) and perhaps subchondral bone marrow lesions (i.e., Modic changes) (see Chapter 11 ) that may be contributing to the pain cascade [ , ]. This chapter discusses the pathophysiology of the endplate, mechanisms that may lead to abnormalities/defects/changes and their clinical implications, and the various classification schemes of the structural endplate phenotype of the spine.
The vertebral endplate during development
From birth until adulthood, the anatomy and composition of the vertebral endplates continually change. Developing from the mesoderm and notochord, the IVDs are positioned between biconvex cartilaginous tissues (see Chapter 1 ). Ossification of the vertebral body takes place during early life. While the outermost rim of the endplate is ossified in young adult spines, the broad central layer remains cartilaginous and is known as the “cartilaginous endplate” (CEP) ( Fig. 10.1 ) [ ]. Ultimately, two zones of the CEP form: the central cartilaginous plate and the peripheral fibrocartilaginous junction with the annulus fibrosus (AF) functioning as an enthesis [ ]. Typical concavity of the endplate starts to appear around the third year of life, almost a year after the establishment of the secondary lumbar curvature in children [ ]. Between the ages of 6 and 8, the epiphyseal cartilage begins to thin, fitting the superior and inferior margins of the vertebral body and consequently serving as a precursor of the annulus/vertebra attachment [ ]. By the age of 12, peripheral ossification centers outside the epiphyseal plate coalesce and form the ring apophysis ( Fig. 10.2 ). Until the ages of 16–21, there remains a layer of hyaline cartilage between the ring apophysis and vertebral body, which contributes to vertebral growth. Thereafter, the ring apophysis begins to fuse to the osseous mass of the vertebral body that signals the cessation of longitudinal growth [ , ].


Structure and composition of the endplate
There are two distinct components of the endplate: bony and cartilaginous. Both components have distinct structural, morphological, and molecular features that are important to the endplate’s overall functions. Intradisc zonal variations from the periphery to the center of the endplate, much like those observed in the IVD, and interdisc heterogeneity along the cranial to caudal axis also exist.
The bony endplate
The bony component of the endplate is a layer of porous, coalesced trabecular bone that forms the boundary between the CEP and cancellous core of the vertebral body. It contains pockets of vascularized marrow, which touch the surface of, and can penetrate a short depth into the CEP. These pockets facilitate the two-way transport of nutrients and cellular metabolic products between the disc and thin-walled vertebral capillaries [ ].
Throughout the spine, central and peripheral heterogeneity of the bony endplate persists.
Generally, the bony endplate is thinner and more porous centrally and thicker peripherally [ , ]. The thicker peripheral section of the endplate is wider anteriorly than posteriorly [ ], occupying a margin of almost 3–7 mm [ ]. The rim of the bony endplate is regarded as the transitional area and junction of the cartilaginous endplate and AF with the vertebral body ( Fig. 10.2 ) [ ]. This is where the annulus/vertebra interface is formed. The interface is an enthesis composed of a fibrocartilaginous composite. Annular fibers are embedded into a zone of calcified cartilage that is anchored to the subchondral bone via geometric interdigitation [ ]. It is evident through microradiographic studies that the outer AF fibers of the adjacent IVDs penetrate through the calcified layer of cartilage to reach attachment sites in the vertebral body. Furthermore, “fibrillary continuity” exists between the AF and calcified region rather than the AF and bone [ ]. This calcified cartilage is crucial for the load transfer between two mechanically dissimilar materials and is reported to exist across all regions of the vertebral endplate (see Chapter 2 ) [ , ]. This is juxtaposed by the fibers of the inner AF, which form a continuity collagen of the CEP ( Fig. 10.3 ) [ ] and ensures a strong union to provide 360-degree containment of the nucleus pulposus (NP) during compression [ ]. In doing so, the CEP may also provide resistance to tensile, shear, and compressive forces during the complex pattern of loading [ ]. The outermost AF fibers merge with the vertebral periosteum, an attachment that helps the disk resist bending loads ( Fig. 10.1 ) [ ].

The cartilaginous endplate
The CEP is a thin layer of hyaline cartilage with an average thickness of 0.50 mm (±0.13 mm) situated between the cranial and caudal ends of the IVDs and the adjacent bony endplates [ ]. It has a base that contains a dense network of capillaries that is formed by terminal branches of metaphyseal and nutrient arteries [ ]. These capillaries exist within bony endplate pores and contain muscarinic receptors that regulate and alter disc blood flow [ , ] in response to external stimuli like nicotine [ , ] or vibration [ ]. The terminal ends of these capillaries form microvascular loops [ ]. This provides increased surface area and therefore better permeability of nutrients into the IVD center.
In adults, the cartilaginous endplate is an avascular structure. By the age of 8–10 years, the immature CEPs of infants and children are believed to be obliterated. It is speculated if this plays a role in inducing interstitial growth of the AF [ , ]. Furthermore, it is hypothesized that the remnants of these vascular canals are structurally weak areas where mechanical stress may cause the herniation of nuclear material into the vertebral body [ ].
Endplate zone variation
In comparison to the inner annulus region, the CEP adjacent to the NP contains much greater complexity in architecture and increased density [ , ]. This zonal variation is also seen in morphological features of the bony endplate, such as its thickness, architecture of the adjacent trabecular network, and its porosity [ , , ]. In comparison to the periphery, the central zone is thinner and more porous [ , ]. These distinctions may offer biologically desirable features that can facilitate the transport of nutrients and metabolites between vertebral capillaries and NP cells. However, this may be structurally undesirable due to compromise of the mechanical strength of the central zone [ ]. The requirements for the endplate to be both porous and strong predicates its structural and compositional complexity [ ].
Along the cranial and caudal axis heterogeneity of the vertebral trabecular structure is also apparent. Compared to the superior vertebra, the inferior vertebral endplate is supported by a stronger and thicker trabecular network [ , , ]. It is likely that these differences cause vulnerable defects and fractures in the cranial endplate [ ].
Cellular and molecular structure
Chondrocytes are distributed throughout the depth of the CEP. They are round and oval in shape and are the principal cells that form the cellular matrix ( Fig. 10.3 ). These cells are essential in the maintenance of the extracellular matrix as they provide stability to the tissue. Furthermore, the density in comparison to the IVD tissue is much higher as cells are observed to be more densely accumulated in the central region of the endplate adjacent to the nucleus [ ]. The cells of the CEP are known to possess similar cytoskeletal structures and filopodia, much like the chondrocytes of articular cartilage. This enables them to migrate toward the NP and also replace notochordal cells in the early stages of life. These chondrocytes exhibit several features that allow them to migrate through tiny pores of 8 μm size. Features such as protrusion of lamellipodia, contraction of cell body, and detachment of the rear to move forward enable movement through the tiny pore [ ]. Migration of these chondrocytes causes thinning of the CEP and further leads to the disappearance of zonal arrangement [ ].
Hyaluronan, proteoglycans, and type VI collagen are the main components of the pericellular matrix around the chondrocytes. Many histochemical studies concerning the hyaline cartilage indicated the occurrence of fibronectin [ , ], laminin [ ], biglycan [ ], and type IX collagen [ , ] within the pericellular matrix. In one study, the collagen content of the CEP was on average 57.5% (±19.2%), varying from central to lateral region in comparison to the bovine cartilaginous endplate with an average of 41.1% (±9.5%) collagen by dry weight in the central region in comparison to 73.8% (±10.8%) in the lateral region [ ]. Type II is the main collagen that is evident in the CEP, while type III, VI, IX, and X also exist in small variable quantities [ , ]. Type III and VI collagens are mainly found in the pericellular regions while type IX is present in the territorial matrix [ , ]. In the mouse model, type II collagen helped to maintain the glycosaminoglycan level within the tissue while also contributing to the strength of the cartilage. Inactivation of a single allele for a type II collagen can produce premature calcification of the endplate with lower levels of proteoglycans in the vertebral endplate and the IVD [ ]. Type IX collagen deficiency induces osteoarthritic changes in the CEP while also encouraging abnormal cellular proliferation, matrix disorganization, and new bone formation in mice [ ]. Another important collagen of the CEP, type X collagen, has also been thoroughly investigated and appears to increase with age, and serves as an important marker of hypertrophic chondrocytes that may play a role in the calcification of cartilage [ ].
Proteoglycan, a protein bonded to a glycosaminoglycan (GAG), is abundant in the extracellular matrix and is required to maintain adequate hydration. Large proteoglycan molecules have an aqueous, gel-like structure that is enmeshed with a dense network of collagen fibrils in its matrix. Recent biochemical analysis of the average GAG content in the CEP of bovine IVD indicates 90.1 mg (±17.8 mg) per mg of dry tissue, which is 20.4% (±3.1%) by dry weight in the center decreasing to 11.7% (±2.1%) in the lateral region. Among the four regions of the CEP, there exist quantitative variations with respect to the center with a significantly higher GAG content than in the lateral, anterior, and posterior regions [ , ]. Proteoglycans of the CEP are also thought to regulate the movement of solutes into and out of the IVD [ ].
The abundance of different types of proteoglycans in the CEP determines the extent of water retention. The proteoglycans of the CEP are rich in keratan sulfate and have a decreased capacity to retain water in comparison to those present in articular cartilage, which are more negatively charged and contain more chondroitin sulfate [ ]. The histology and biochemical composition of degeneration, spinal levels, and depth within the endplate [ ] differs with age and further confers varying physical properties [ ]. Close to the discochondral junction, the CEP has a reduced amount of collagen while remaining rich in proteoglycans and water in comparison to the rest of the cartilaginous tissue which includes its interface with the bony endplate [ , ].
Endplate function
The endplate transfers mechanical loads at the soft/hard interface [ , ] serving as a critical function in the upkeep and health of the spinal column while also maintaining a major route of transportation between IVD cells and vertebral body capillaries. This is especially necessary at the central point of the IVD [ ]. The endplate structure and composition must therefore balance conflicting requirements between having resistance to high compressive loads while also allowing sufficient permeability to allow biochemical transport [ ].
Mechanical load transfer
Between the vertebral bodies and the IVDs, the bony and CEPs work in congruence to transfer mechanical loads. The calcified layer of cartilage enables the outer AF fibers to efficiently transmit high tensile loads to the vertebrae [ ], which is a result of graded material properties across this interface. At the central endplate, the CEP is a soft and resilient tissue. Both Type II collagen and proteoglycan arrange in dense matrix are needed to withstand both tensile and compressive loading [ , ]. The typical arrangement of the collagen fibrils within the CEP resists nuclear pressurization during compressive spinal loading (analogous to a drum head), which provides the tissue with tangential strength [ ]. Tensile properties are largely defined by the ratio of collagen to glycosaminoglycan [ ], while compressive properties are reliant on hydrophilic proteoglycans with negatively charged sulfate and carboxyl groups that exert a swelling pressure [ ]. The lateral part of the CEP is stiffer enabling it to share a large proportion of the pressure and load [ ]. Therefore, the structural properties of the vertebral endplates provide resistance to high hydrostatic pressure, which is generated in the NP. The vertebral endplate maintains a uniform distribution of compressive load between the IVD and the vertebral body while simultaneously providing the AF with sufficiently strong attachments needed to resist spinal bending [ ].
Biochemical transport
The IVD is avascular and hence disc cells are highly dependent on transport through the CEP for their viability and to further synthesize and maintain extracellular matrix [ ]. Depending on the size of the solute, the molecules will either reach IVD cells through diffusion or convection. [ ]. Generally, smaller solutes such as glucose, lactate, sulfate, and oxygen travel through diffusion [ ] while larger elements are transported via convection [ , ]. Dynamic spinal loading is thought to enhance transport and improve IVD cell function as pressure drives convection [ ].
Using microsphere and electron microscopic studies, vertebral endplate capillaries have been mapped [ , ]. In the central region [ , ], the blood vessels are most dense [ ]; studies have shown that capillary buds are coiled to increase surface area for permeability. It is selectively permeable because the CEP matrix structure and charge allows smaller, neutral molecules and small cations to pass easily through the matrix. Charged proteoglycans hinder anion exchange to a certain extent [ ]. Diurnal mechanical loading is a big influencing factor [ , ], as certain growth factors, enzymes, and various other large solutes move in and out of the nucleus by convection or fluid flow. Experiments on animal models illustrate that the rate of cyclic loading also affects small molecule transport and is different for healthy and degenerated IVDs [ ]. There may be a reduction in convection and permeability of different solutes due to compressive loading that compacts the CEP matrix [ , ].
The CEP acts as a chemical and biological filter between the vertebral capillaries and nucleus tissue, the permeability of which is dependent on its composition. With age and degeneration, CEP composition undergoes changes such as alterations in calcium, proteoglycan and collagen,that together influence its hydration and pore size; thus, reducing the permeability. Calcification of the endplate, a common feature of aging, degeneration, and scoliosis, has been reported as a consequence of a reduction in diffusivity of the CEP [ , , ]. This strongly affects glucose penetration and permeability resulting in the reduced level of nutrients in the IVD, most prominently in the NP [ , ]. Additionally, abnormal diffusion patterns produced by breaks or damage allows cytokines, bacteria, and inflammatory cells to move freely through this path ( Fig. 10.4 ) [ , ].

Endplate and back pain
The vertebral endplate is a highly innervated structure that designates it as a potential pain-generating source, in particular, if it sustains damage or structural change [ , , ]. Various forms of vertebral endplate damage or defect, such as Schmorl’s nodes, erosions, fractures ( Fig. 10.5 ) and calcifications, have been and associated with IVD degeneration and low back pain ( Fig. 10.6 ) [ ]. However, some studies question whether vertebral endplate damage associates with back pain, especially since such imaging phenotypes (e.g., Schmorl’s nodes) may exist in asymptomatic individuals [ , ]. For example, in a large-scale study of twin volunteers, it was noted that Schmorl’s nodes, while related to IVD degeneration, were not themselves an independent risk factor for back pain [ ]. It is not surprising, therefore, that numerous other studies offer conflicting evidence regarding the role of the specific vertebral endplate phenotypes with spine-related pathology and symptom development linking endplate change with IVD degeneration and pain [ , , ]. It is likely that some forms of endplate damage are more prone to trigger symptoms than others. For example, some studies using provocative discography indicate that endplate degeneration can be a potential source of back pain [ , , ]. Yet, others suggest that endplate degeneration should not be considered as an immediate or primary cause of pain [ ] and rather considered “a sequelae of disc degeneration.” Population-based and twin studies have also noted that endplate changes may potentially predict future disc degeneration [ ]. In addition, various studies have noted that preexisting endplate abnormalities/changes/defects may predict adjacent segment degeneration following spine fusion, further underscoring the clinical relevance of the endplate in predicting outcomes following spine treatment [ , ].
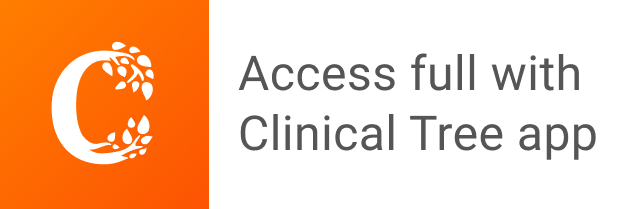