Introduction
Ultrasound guidance can be applied to assist in a multitude of interventional orthopedic procedures. This chapter provides a review of ultrasound physics, image optimization, common artifacts, and fundamentals of ultrasound-guided interventions.
Ultrasound Principles
Diagnostic ultrasound knowledge complements interventional ultrasound skills. Understanding ultrasound physics, image optimization, and artifacts are key components of diagnostic ultrasound. Mastery of diagnostic ultrasound serves as the foundation for safe and effective ultrasound-guided interventions. Awareness of sonographic artifacts and why these occur is imperative to distinguish those artifacts from pathology and to effectively prevent or mitigate artifacts that could make an intervention unsafe or more challenging.
Ultrasound Physics
A comprehensive description of the pertinent physics of ultrasound mechanics and the resulting imaging output is beyond the scope of this chapter. Briefly, electric current is transmitted from a wall-plug or an affixed battery through the ultrasound processor and, ultimately, to the ultrasound transducer. The head of an ultrasound transducer contains an array of piezoelectric crystals, which convert the electric current into pulses of ultrasonic waves. The piezoelectric crystal array within a transducer head can vary in the alignment, shape, thickness, and width, which influence quality of spatial resolution, the depth of ultrasound wave penetration, and signal-to-noise ratio. The transducer is coupled to the skin of the patient through a sonoconductive gel for sound waves to conduct through the target tissue.
Once the sound wave travels through patient’s skin, the energy of the waves can be reflected by a tissue, absorbed by a tissue, or penetrate through a tissue. More dense tissues will reflect more ultrasound energy, and less dense tissues will permit greater penetration of ultrasound energy. As the sound waves penetrate deeper, energy is lost to refracted and absorbed sound waves and the ultrasound signal becomes attenuated or weaker in strength. Reflected sound waves travel retrograde to return to the transducer where they interact with the piezoelectric crystal array of the transducer head. Those reflected sound waves are converted back to an electrical signal, by a reverse piezoelectric effect, and are transmitted to the central processor of the ultrasound machine. The processor knows the pulse velocity of the anterograde ultrasound waves that were transmitted and can calculate the depth of reflecting structures from the measured echo time to create an ultrasound image. Bright, hyperechogenic-appearing structures result from the reflection of greater amounts of ultrasound energy by more dense tissues, which have higher impedance, such as bone. Conversely, dark, hypoechogenic-appearing structures result from the reflection of less amount of ultrasound energy by less dense tissues with lower impedance, such as fibrocartilage.
Image Optimization
Image optimization requires selection of the most appropriate ultrasound transducer and skillful manipulation of ultrasound probe and ultrasound machine settings such as depth, focal zone number and location, gain, and dynamic range. This is imperative to best visualize the targeted structure, to permit the most complete visualization of the needle or tool, and to ensure the safety and avoidance of neurovascular structures.
Transducer Selection
Ultrasound transducers vary by the range of ultrasound frequencies (labeled on the body of the transducer in megahertz [MHz]) that they transmit and the size of the transducer head footprint ( Fig. 2.1 ). In general, a linear array transducer can transmit higher-frequency, lower-amplitude ultrasound waves. The higher-frequency transducer will transmit more ultrasound waves and thus receive more returning ultrasound waves, facilitating the production of greater detail and spatial resolution in the image produced. However, the lower-amplitude waves have less energy and will become attenuated and lose strength more quickly, so deeper objects are not as well visualized. In contrast, a curvilinear array transducer will transmit lower-frequency, higher-amplitude waves. The lower-frequency transducer will transmit fewer ultrasound waves, but those waves will have a higher amplitude and more energy; thus they are less susceptible to attenuation as they penetrate to deeper tissues. As a result, more sound waves return from deeper tissues and deeper objects are better visualized, but there is less detail and spatial resolution of superficial tissues.

In general, a lower-frequency curvilinear array transducer is preferable for tissues targeted at 4 to 5 cm and deeper and a higher-frequency linear array transducer will be preferable for tissues more superficial than that. The convex transmission of ultrasound waves from a curvilinear array transducer can improve visualization of a needle directed at a steep angle. The curvilinear array directs more ultrasound waves perpendicular to that needle that are reflected at an angle of incidence that facilitates return to the transducer, improving needle visualization. This same benefit can be obtained in beam-steering mode on linear array transducers, which is described later in this chapter.
Specialized small-footprint linear array transducers (commonly referred to as “hockey-stick” transducers because of their shape) will have a smaller footprint of approximately 15 to 20 mm (vs. 45+ mm for a standard linear transducer), which can facilitate improved skin contact and beam coupling on the sharp contours of the hands and feet. The smaller footprint also decreases the size of the field of view, which must also be considered when performing interventional procedures.
Knobology
The layout of knobs, switches, and touch screen interfaces will vary by ultrasound platform manufacturer and by different models within a manufacturer’s range of platforms. It is essential for each individual operator to familiarize himself or herself with the layout of the control panel of each platform that might be used. The depth of the ultrasound image should be adjusted, often by turning a dial or adjusting a switch up or down, to ensure that the targeted structure and any “at-risk” structures are captured but also that the image does not have unnecessary depth beyond those structures ( Fig. 2.2 ).

Focal zone depth can be adjusted and their number increased or decreased on most platforms. Focal zones represent the narrowest segments of the ultrasound beam where the ultrasound processor is directed to optimize the ultrasound waves and processing power so as to produce the best temporal and spatial resolution ( Fig. 2.3 ). The number and positioning of focal zones should be modified according to the depth and size of the targeted structure. Minimization of the number of focal zones reduces processing demands by reducing the frame rate and improves image resolution.

Gain increases or decreases the overall brightness of the raw returned echoes and is most often adjusted and increased to account for beam attenuation resulting in image hypoechogenicity. Increasing or decreasing gain with the dial or switch does so uniformly and modifies the entire image. Time gain compensation (TGC) involves the individual manipulation of one of a vertically oriented stack of sliding switches which correlate to different image depths. Modification of the TGC permits focal manipulation of gain at specific image depths ( Fig. 2.4 ). Typically, the TGC is used to focally increase gain in the far field to account for beam attenuation and relatively hypoechogenic imaging at increasing depths.

Sonographic Appearance of Normal Structures
An ultrasound image is produced by the return and processing of ultrasound waves by the transducer, which is affected by how the ultrasound waves penetrate, reflect, or are absorbed by different tissues. Different image echogenicities represent the reflection of waves at the interface of structures of relatively different density and orientation within a single tissue type or between different adjacent structures ( Fig. 2.5 ). The interface between structures with a wide difference in impedance (e.g., that seen between soft tissue and bone) results in more reflected sound waves and producing a relatively bright, or hyperechoic, structure on the ultrasound image . Structures that are less dense or sit adjacent to a very dense structure will reflect fewer sound waves or waves with smaller amplitude, resulting in a less bright, or hypoechogenic-appearing, structure. Adjacent structures of similar impedance are termed isoechoic to each other. Regions where all ultrasound waves are absorbed and no echo returns to the transducer appear black on an ultrasound image and are termed anechoic .


Comprehensive knowledge of local and regional musculoskeletal and neurovascular anatomy is essential. Tendon, ligament, muscle, bone, cartilage, bursa, and peripheral nerve have distinct sonographic appearances ( Table 2.1 ). A thorough understanding of the normal sonographic appearance and surrounding anatomy of pertinent structures in both short-axis and long-axis imaging planes is critical to identify potentially at-risk structures, recognize congenital variation or absence of a structure, and diagnose pathology. , A comprehensive description of the sonographic appearance of pathologic tissues and anatomic variations is beyond the scope of this text.
Structure | Appearance on Ultrasound |
---|---|
Tendon | Linearly oriented between a muscle body and osseous insertion. Homogeneously and densely oriented fibers produce a dense, linear hyperechoic structure in long-axis imaging with distinct superficial and deep tenosynovial borders. In short axis, tendon appears as a regular-bordered ovoid structure with homogeneously echogenic and sized internal tendon fibers, often referred to as a “broomstick” viewed on end ( Fig. 2.6 ) |
Ligament | Linearly oriented between two osseous structures. Ligament is composed of homogeneously and densely oriented fibers and visualized as echogenic fibrillar fibers, which tend to be less echogenic than tendons but echogenicity also depends on anisotropy and contrast with surrounding structures. Although the echotexture of nonpathological ligaments has not been well described, they are often visualized as a linear hyperechoic structure in long-axis imaging. In short axis, ligament will appear as a more irregularly bordered, flattened structure |
Muscle | Composed of relatively hypoechoic muscle fibers and interspersed hyperechoic intramuscular stromal connective tissue of the endomysium and perimysium. In short axis, its appearance is often referred to as a “starry night” |
Bone | Densely hyperechoic relative to all surrounding tissues because of the inherent high acoustic impedance mismatch. Cortical bone appears as a continuous, regular, brightly hyperechoic line with deep reverberation artifact and posterior acoustic shadowing which precludes visualization of any deeper structures |
Hyaline cartilage | Densely and evenly hypoechoic secondary to its high water content and the high acoustic impedance mismatch with the deep bone |
Fibrocartilage | Homogeneously hyperechoic relative to more superficial connective tissue or muscle. It has the same appearance in both long- and short-axis orientations |
Bursa | When distended with fluid, anechoic-to-mixed-echogenic fluid and debris can fill the bursa. Firm transducer pressure will compress and displace the fluid |
Peripheral nerve | Hyperechoic perineurium and endoneurium, which surrounds numerous small, round, and hypoechoic nerve fascicles. All of this is contained within a hyperechoic epineurium, giving a “honeycomb” appearance when visualized in short axis (see Fig. 2.6 ) |
Sonographic Artifacts
Ultrasound imaging is inherently susceptible to image artifacts because the sonographic character of normal tissue can change based on the angle of insonation of the ultrasound beam and the relative sonographic characteristics of adjacent tissues. A thorough awareness of such artifacts and an understanding of why they occur is essential to avoid erroneous diagnosis of pathology and unnecessary and unproductive procedures. Common artifacts include anisotropy, shadowing, posterior acoustic enhancement, posterior reverberation, and beam-width artifact.
Anisotropy
Anisotropy is the artifactually hypoechoic or anechoic appearance of a structure that occurs when a structure is imaged at an angle of incidence. The angle of incidence is the angle at which the ultrasound waves encounter the surface of a structure. If the angle is perpendicular, or close to 90 degrees, more waves will be reflected back to the transducer. If the ultrasound waves are more parallel, waves will be reflected or “scattered,” resulting in a failure of the anticipated ultrasound waves returning to the transducer head. Anisotropy will occur when imaging structures in both long and short axes. Tendons and ligaments are most susceptible, specifically when curving around a bony prominence or quickly changing depth to become more deep or superficial ( Fig. 2.7 ). Anisotropy produces an artifactually hypoechoic appearance that can mimic pathology.

Anisotropy can be minimized or eliminated in short-axis visualization by angulating or “wagging the tail” of the transducer to ensure that the ultrasound beam is perpendicular to the structure (face of the probe is parallel to the structure), approximating the angle of incidence as close to 90 degrees as possible. When visualizing a structure in long axis, anisotropy is addressed by heel-toeing or “rocking” one end of the transducer to ensure that the ultrasound beam is perpendicular to the structure, again, approximating the angle of incidence as close to 90 degrees as possible.
Posterior Acoustic Shadowing
Posterior acoustic shadowing results when ultrasound waves are reflected or attenuated by a structure resulting in little, to no, waves penetrating through to deeper structures ( Fig. 2.8A ). This results in a relatively hypoechoic appearance of all tissues deep to the structure. Dense structures, such as bone, calcifications, and foreign bodies, are most likely to cast a posterior acoustic shadow.

Posterior Acoustic Enhancement
Posterior acoustic enhancement, or increased through-transmission, occurs deep to structures that are hypoechoic relative to adjacent tissues, resulting in less ultrasound beam attenuation or reflection (see Fig. 2.8B ). Tissues deep to the less dense structure will appear relatively hyperechoic compared with adjacent soft tissues because relatively more of the ultrasound waves penetrate through the more superficial and less absorptive structure to the deeper tissues. This artifact can be used to advantageously image structures deep to vasculature and cystic or fluid-filled structures.
Reverberation Artifacts
Reverberation appears as a series of hyperechoic, linear artifacts deep to dense structures and results from a series of ultrasound wave reflections between two parallel, highly reflective surfaces. The single reflection will be displayed at the proper location but the artifactual late return of attenuated, reflected ultrasound waves are interpreted by the ultrasound processor as deeper, hypoechoic structures. This commonly occurs with bone and with metal surfaces, such as a needle or orthopedic implant ( Fig. 2.9 ). Ring-down artifact appears as a solid streak or series of parallel bands which result from the resonant vibration of air bubbles. Comet-tail artifact appears as a series of multiple closely spaced reverberation echoes deep to a more focal or punctate structure which results from sequential echoes from two closely spaced, highly reflective interfaces.

Beam-Width Artifact
Beam-width artifact results when the ultrasound beam is too wide relative to a small object being imaged and is similar to volume averaging in magnetic resonance imaging (MRI). For example, shadowing from a small calcification may not be visualized due to a wide beam width. This artifact can be eliminated by adjusting the focal zone to the level of the object of interest or changing to a probe with a smaller footprint.
Visualization of Blood Flow
Color and power Doppler imaging detect motion toward or away from the transducer by detecting the delay between frequencies of the transmitted and received ultrasound waves ( Fig. 2.10 ). , Color Doppler displays differences in flow direction, red color representing flow toward the transducer and blue color representing flow away from the transducer. Power Doppler does not discriminate direction of flow but is more sensitive to low flow and provides superior detection of small vessels and slow flow rates. Power Doppler is sensitive to transducer movement and susceptible to flash artifact. Increased blood flow on Doppler imaging may occur with greater perfusion, inflammation, and neovascularization and can assist in differentiating complex fluid and synovitis because the former will often lack blood flow whereas the latter demonstrates increased flow.

Microvascular imaging mode, such as superb microvascular imaging (SMI), has been demonstrated to have greater sensitivity for the identification of microvessels at a high frame rate. This technology facilitates detailed visualization of microvessels and lower-velocity blood flow without the use of contrast medium.
Role for Ultrasound Guidance in Interventional Orthopedic Procedures
General indications for needle placement into or about a soft tissue or joints include, but are not limited to, the removal of fluid for diagnostic evaluation or symptom palliation and the delivery of diagnostic or therapeutic agents. When considering the use of image guidance for a procedure, one should understand the indications and contraindications. Ultrasound affords many distinct advantages as an imaging modality for procedure guidance, but clinicians should be able to discern when the inherent limitations of ultrasound may make another imaging modality a safer or more effective tool for the procedure.
Advantages of Ultrasound
The use of ultrasound as an imaging modality to assist in the guidance of musculoskeletal interventions has the potential to improve accuracy, efficacy, and safety when compared with palpation guidance. , When used for procedural guidance, ultrasound also affords multiple distinct advantages over x-ray, MRI, and computed tomography (CT) that make it an ideal diagnostic and interventional imaging tool ( Table 2.2 ). Ultrasound can be used at the point of care to identify tendinosis/tendinopathy, ligamentous injury, muscular injury, bony pathology and joint pathology (i.e., effusion, hemarthrosis, loose body, meniscal injury). Clinical evaluation, diagnostic ultrasound evaluation, and progression to an ultrasound-guided intervention are possible to complete in a single outpatient clinic visit.
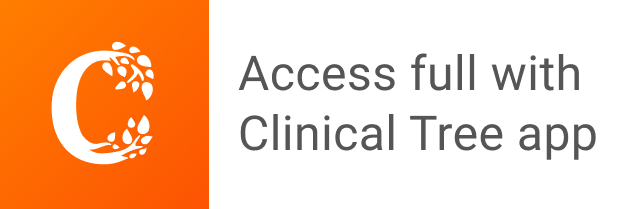