General Principles
Regular physical activity is an important component of a healthy lifestyle. Increases in physical activity and cardiorespiratory fitness have been shown to reduce the risk for death from coronary heart disease as well as from all causes. The primary focus on achieving these health-related goals in the past has been on prescribing exercise to improve cardiorespiratory fitness, body composition, and strength. Recently, the Centers for Disease Control and Prevention (CDC) and the American College of Sports Medicine (ACSM) suggested that the focus be broadened to address the needs of more sedentary individuals, especially those who cannot or will not engage in structured exercise programs. There is increasing evidence showing that regular participation in moderate-intensity physical activity is associated with health benefits, even when aerobic fitness remains unchanged. To reflect this evidence, the CDC and ACSM are now recommending that every adult in the United States accumulates 30 minutes or more of moderate-intensity physical activity on most, and preferably all, days of the week. Those who follow these recommendations can experience many of the health-related benefits of physical activity, and if they are interested are ready to achieve higher levels of fitness.
Important in prescribing exercise is an understanding of the principles of specificity and periodization. The principle of specificity states that metabolic responses to exercise occur most specifically in those muscle groups being used. Furthermore, the types of adaptation will be reflective of the mode and intensity of exercise. The principle of periodization reflects the importance of incorporating adequate rest to accompany harder training sessions. Overall training programs (macrocycles) are divided into phases (microcycles), each with specific desired effects (i.e., enhancing a particular energy system or sport-specific goal).
This chapter provides a brief overview of the basic fundamentals of exercise physiology, including the metabolic energy systems, and the basic muscle and cardiorespiratory physiology associated with exercise. It will then provide an overview of the exercise prescription according to the current ACSM guidelines, and the fundamentals of exercise programming, including preexercise screening.
Energy Systems
A 70-kg human has an energy expenditure at rest of approximately 1.2 kcal/min, with less than 20% of the resting energy expenditure attributed to skeletal muscle. During intense exercise, however, total energy expenditure can increase 15 to 25 times above resting values, resulting in a caloric expenditure between 18 and 30 kcal/min. Most of this increase is used to provide energy to the exercising muscles that can increase energy requirements by a factor of 200.
The energy used to fuel biological processes comes from the breakdown of adenosine triphosphate (ATP), specifically from the chemical energy stored in the bonds of the last two phosphates of the ATP molecules. When work is performed, the bond between the last two phosphates is broken, producing energy and heat:
ATP → ATPase ADP + Pi + energy
The limited stores of ATP in skeletal muscles can fuel approximately 5 to 10 seconds of high-intensity work ( Figure 15-1 ). ATP must be continuously resynthesized from adenosine diphosphate (ADP) to allow exercise to continue. Muscle fibers contain three metabolic pathways for producing ATP: the creatine phosphate system, rapid glycolysis, and aerobic oxidation.

Creatine Phosphate System
When limited stores of ATP are nearly depleted during high-intensity exercise (5 to 10 seconds), the creatine phosphate system transfers a high-energy phosphate from creatine phosphate to rephosphorylate ATP from ADP:
ADP + creatine phosphate → creatine kinase ATP + creatine
Because it involves a single reaction, this system can provide ATP at a very rapid rate. Because there is a limited supply of creatine phosphate in the muscle, however, the amount of ATP that can be produced is also limited.
There is enough creatine phosphate stored in skeletal muscle for approximately 25 seconds of high-intensity work (see Figure 15-1 ). The ATP–creatine phosphate system lasts for approximately 30 seconds (5 seconds for the stored ATP and 25 seconds for creatine phosphate). This provides energy for activities such as sprinting and weightlifting. The creatine phosphate system is considered an anaerobic system because oxygen is not required.
Rapid Glycolysis (Lactic Acid System)
Glycolysis uses carbohydrates primarily in the form of muscle glycogen as a fuel source. When glycolysis is rapid, the pathways that normally use oxygen to make energy are circumvented in favor of other, faster yet less efficient paths that do not require oxygen. As a result, only a small amount of ATP is produced anaerobically, and lactic acid is produced as a byproduct of the reaction.
For many years, lactic acid was considered to be the waste product caused by inadequate oxygen supply. Lactic acid limited physical activity by building up in muscles and leading to fatigue and diminished performance. Since the early 1980s, there has been a fundamental change in thought, and evidence now shows that a limited oxygen supply is not required for lactic acid production. Lactate is produced and used continuously under fully aerobic conditions. This is referred to as the cell-to-cell lactate shuttle in which lactate serves as a metabolic intermediate tying together glycolysis (as an end product) and oxidative metabolism.
Once lactic acid is formed, there are two possible venues it can take. The first involves conversion into pyruvic acid and subsequently into energy (ATP) under aerobic conditions (see section on “ Aerobic Oxidation System ”). The second involves hepatic gluconeogenesis using lactate to produce glucose, which is known as the Cori cycle.
Anaerobic oxidation starts as soon as high-intensity exercise begins and dominates for approximately 1.5 to 2 minutes (see Figure 15-1 ). It would fuel activities such as middle-distance sprints (400-, 600-, and 800-m runs) or events requiring sudden bursts of energy, such as weightlifting.
Although glycolysis is considered an anaerobic pathway, it can readily participate in the aerobic metabolism when oxygen is available and is considered the first step in the aerobic metabolism of carbohydrates.
Aerobic Oxidation System
The final metabolic pathway for ATP production combines two complex metabolic processes: the Krebs cycle and the electron transport chain. The aerobic oxidation system resides in the mitochondria. It is capable of using carbohydrates, fat, and small amounts of protein to produce energy (ATP) during exercise, through a process called oxidative phosphorylation. During exercise, this pathway uses oxygen to completely metabolize the carbohydrates to produce energy (ATP), leaving only carbon dioxide and water as byproducts. The aerobic oxidation system is complex and requires 2 to 3 minutes to adjust to a change in exercise intensity (see Figure 15-1 ). It has an almost unlimited ability to regenerate ATP, however, limited only by the amount of fuel and oxygen that is available to the cell. Maximal oxygen consumption, also known as , is a measure of the power of the aerobic energy system, and is generally regarded as the best indicator of aerobic fitness.
All the energy-producing pathways are active during most types of exercise, but different exercise types place greater demands on different pathways. The contribution of the anaerobic pathways (creatine phosphate system and glycolysis) to exercise energy metabolism is inversely related to the duration and intensity of the activity. The shorter and more intense the activity, the greater the contribution of anaerobic energy production, whereas the longer the activity and the lower the intensity, the greater the contribution of aerobic energy production. In general, carbohydrates are used as the primary fuel at the onset of exercise and during high-intensity work. However, during prolonged exercise of low to moderate intensity (longer than 30 minutes), a gradual shift from carbohydrate toward an increasing reliance on fat as a substrate occurs. The greatest amount of fat use occurs at approximately 60% of maximal aerobic capacity ( ).
Cardiovascular Exercise
Cardiorespiratory Physiology
The cardiorespiratory system consists of the heart, lungs, and blood vessels. The purpose of this system is the delivery of oxygen and nutrients to the cells, as well as the removal of metabolic waste products to maintain the internal equilibrium.
Cardiac Function
Heart Rate
Normal resting heart rate (HR) is approximately 60 to 80 beats/min. HR increases in a linear manner with the work rate and oxygen uptake during exercise. The magnitude of HR response is related to age, body position, fitness, type of activity, the presence of heart disease, medications, blood volume, and environmental factors such as temperature and humidity. HR during maximal exercise can exceed 200 beats/min, depending on the individual’s age and training state. With the onset of dynamic exercise, HR increases in proportion to the relative workload. The maximal HR (HR max ) decreases with age, and can be estimated in healthy men and women with the following formula: HR max = 220 − age. There is considerable variability in this estimation for any fixed age, with a standard deviation of ±10 beats/min.
Stroke Volume
Stroke volume (SV) is the amount of blood ejected from the left ventricle in a single beat. SV is equal to the difference between end-diastolic volume and end-systolic volume. Greater diastolic filling (preload) will increase SV. Factors that resist ventricular outflow (afterload) will result in a reduced SV.
SV is greater in men than in women. At rest in the upright position, it generally ranges from 60 to 100 mL/beat, whereas maximal SV approximates 100 to 120 mL/beat. During exercise, SV increases in a curvilinear relationship with the work rate until it reaches near maximal at a level equivalent to approximately 50% of aerobic capacity. SV starts to plateau, and further increases in workload do not result in increased SV, primarily because of reduced filling time during diastole.
SV is also affected by body position, with SV being greater in the supine or prone position and lower in the upright position. Static exercise (weight training) can also cause a slight decrease in SV because of increased intrathoracic pressure.
Cardiac Output
Cardiac output (Q) is the amount of blood pumped by the heart each minute. It is calculated by the following formula:
Q ( L / min ) = HR ( beats / min ) × SV ( mL / beat )
Resting cardiac output in both trained and sedentary individuals is approximately 4 to 5 L/min, but during exercise the maximal cardiac output can reach 20 L/min. Maximum cardiac output in an individual depends on many factors, including age, posture, body size, presence of cardiac disease, and physical conditioning. During dynamic exercise, cardiac output initially increases with increasing exercise intensity by increases in SV and HR. Increases in cardiac output initially beyond 40% to 50% of , however, are accounted for only by increases in HR.
Blood Flow
At rest, 15% to 20% of the cardiac output is distributed to the skeletal muscles, with the remainder going to visceral organs, the brain, and the heart. During exercise, 85% to 90% of the cardiac output is selectively delivered to working muscles and shunted away from the skin and splanchnic vasculature. Myocardial blood flow can increase four to five times with exercise, whereas blood supply to the brain is maintained at resting levels. The difference between the oxygen content of arterial blood and the oxygen content of venous blood is termed the arteriovenous oxygen difference. It reflects the oxygen extracted from arterial blood by the tissues. The oxygen extraction at rest is approximately 25%, but at maximal exercise the oxygen extraction can reach 75%.
Venous Return
Venous return is maintained or increased during exercise by the following mechanisms :
- •
Contracting skeletal muscle acts as a “pump” against the various structures that surround it, including deep veins, forcing blood back toward the heart.
- •
Smooth muscle around the venules contracts, causing venoconstriction. This increases the pressure on the venous side, maintaining blood flow toward the heart.
- •
Diaphragmatic contraction during exercise creates lowered intrathoracic pressure, facilitating blood flow from the abdominal area and lower extremities.
Blood Pressure
Blood pressure is the driving force behind blood flow. Systolic blood pressure (SBP) is the maximal force of the blood against the walls of the arteries when cardiac muscle is contracting (systole). Normal resting SBP is less than 130 mm Hg. Diastolic blood pressure (DBP) is the force of the blood against the walls of the arteries when the heart is relaxing (diastole). Normal resting DBP is less than 85 mm Hg.
SBP increases linearly with increasing work intensity, at 8 to 12 mm Hg per metabolic equivalent (MET) (where 1 MET = 3.5 mL of O 2 per kilogram per minute). Maximum values typically reach 190 to 220 mm Hg. Maximum SBP should not be greater than 260 mm Hg. DBP remains unchanged or only slightly increases with exercise.
Because blood pressure is directly related to cardiac output and peripheral vascular resistance, it provides a noninvasive way to monitor the inotropic performance (pumping capacity) of the heart. Failure of SBP to rise, decreased SBP with increasing work rates, or a significant increase in DBP are all abnormal responses to exercise, and indicate either severe exercise intolerance or underlying cardiovascular disease.
Postural Considerations
In the supine position, gravity has less effect on return of blood to the heart, thus the SBP is lower. When the body is upright, gravity works against the return of blood to the heart, thus SBP increases. DBP does not change significantly with body position in healthy individuals.
Effects of Arm and Leg Exercises
At similar oxygen consumptions, HR, SBP, and DBP are higher during arm work than during leg work. This is primarily because the total muscle mass in the arms is smaller, and consequently a greater percentage of the available mass is recruited to perform the work. In addition, arm work is less mechanically efficient than leg work.
Pulmonary Ventilation
Pulmonary ventilation ( ) is the volume of air exchanged per minute, and generally is approximately 6 L/min at rest in an average sedentary adult man. However, at maximal exercise,
increases 15- to 25-fold over resting values. During mild to moderate exercise,
increases primarily by increasing tidal volume, but during vigorous activity it increases by increasing the respiratory rate.
Increases in are generally directly proportional to an increase in oxygen consumption (
) and carbon dioxide that is produced (
). At a critical exercise intensity (usually 47% to 64% of the
in healthy untrained individuals and 70% to 90%
in highly trained individuals), however,
increases disproportionately relative to the
(paralleling an abrupt increase in serum lactate and
). This is called the anaerobic (ventilatory) threshold.
Anaerobic Threshold
The anaerobic threshold signifies the onset of metabolic acidosis during exercise, and traditionally has been determined by serial measurements of blood lactate. It can be noninvasively determined by assessment of expired gases during exercise testing, specifically and carbon dioxide production (
). The anaerobic threshold signifies the peak work rate or oxygen consumption at which the energy demands exceed the circulatory ability to sustain aerobic metabolism.
Maximal Oxygen Consumption
The most widely recognized measure of cardiopulmonary fitness is the aerobic capacity, or . This variable is defined physiologically as the highest rate of oxygen transport and use that can be achieved at maximal physical exertion.
The resting oxygen consumption of an individual (250 mL/min) divided by body weight (70 kg) gives the resting energy requirement, 1 MET (approximately 3.5 mL/kg per minute). Multiples of this value are used to quantify levels of energy expenditure. For example, running a 6-mph pace requires 10 times the resting energy expenditure, giving an aerobic cost of 10 METs, or 35 mL/kg per minute. Because there is little variation in HR max and maximal systemic arteriovenous oxygen difference with physical training, virtually defines the pumping capacity of the heart. When expressed as milliliters of oxygen per kilogram of body weight per minute (mL/kg per minute) or in METs, it is considered the best index of physical work capacity or cardiorespiratory fitness.
Oxygen Pulse
The oxygen pulse (mL/beat) is the ratio of (mL/min) to HR (beats/min), when both measures are obtained simultaneously. Oxygen pulse increases with increasing work effort. A low value during exercise indicates an excessive HR for workload and can be an indicator of heart disease.
Respiratory Quotient and Respiratory Exchange Ratio
The respiratory quotient (RQ) is the ratio of CO 2 produced by cellular metabolism to O 2 used by tissues. It quantifies the relative amounts of carbohydrate and fatty acids being oxidized for energy. An RQ of 0.7 implies dependence on free fatty acids. An RQ of 1.0 indicates dependence on carbohydrate. The RQ does not exceed 1.0.
The respiratory exchange ratio (RER) reflects pulmonary exchange of CO 2 and O 2 at rest and during exercise. The RER also ranges between 0.7 and 1.0 during rest, and can also reflect substrate preference. During strenuous exercise, however, the RER can exceed 1.0 because of increasing metabolic activity not matched by and additional CO 2 derived from bicarbonate buffering of lactic acid. The terms respiratory quotient and respiratory exchange ratio are often used interchangeably, but their distinction is important.
Effects of Exercise Training
Cardiovascular System
The effects of regular exercise on cardiovascular activity can be grouped into changes that occur at rest, during submaximal exercise, and during maximal work ( Box 15-1 ). Regular exercise can also affect a number of physiologic measurements ( Box 15-2 ).
Changes at Rest
- •
Heart rate decreases, probably secondary to decreased sympathetic tone, increased parasympathetic tone, and a decreased intrinsic firing rate of the sinoatrial node.
- •
Stroke volume increases secondary to increased myocardial contractility.
- •
Cardiac output is unchanged at rest.
- •
Oxygen consumption does not change at rest. *
* From Rupp JC: Exercise physiology. In Roitman JL, Bibi KW, Thompson WR, editors: ACSM health fitness certification review, Philadelphia, 2001, Lippincott Williams & Wilkins; Seto C: Basic principles of exercise training. In O’Connor F, Sallis R, Wilder R, et al, editors: Sports medicine: just the facts, New York, 2005, McGraw-Hill.
Changes at Submaximal Work †
† Submaximal work is defined as a workload during which a steady state is achieved.
- •
Heart rate decreases, at any given workload, because of the increased stroke volume and decreased sympathetic drive.
- •
Stroke volume increases because of increased myocardial contractility.
- •
Cardiac output does not change significantly because the oxygen requirements for a fixed workload are similar. The same cardiac output is generated, however, with a lower heart rate and higher stroke volume.
- •
Submaximal oxygen consumption does not change significantly because the oxygen requirement is similar for a fixed workload.
- •
Arteriovenous oxygen (a-v o 2 ) difference increases during submaximal work.
- •
Lactate levels are decreased because of metabolic efficiency and increased lactate clearance rates. *
Changes at Maximal Work
- •
Maximum heart rate does not change with exercise training.
- •
Stroke volume increases because of increased contractility and/or increased heart size.
- •
Maximal cardiac output increases because of increased stroke volume.
- •
Maximal oxygen consumption (
) increases primarily because of increased stroke volume.
- •
Ability of the local mitochondria to use oxygen is improved. * (Because measured a-v o 2 difference represents whole-body a-v o 2 difference, there is generally little change in the measured a-v o 2 difference.)
Blood Pressure
- •
In normotensive individuals, regular exercise does not appear to have a significant impact on resting or exercising blood pressure. In individuals with hypertension, there can be a modest reduction in resting blood pressure as a result of regular exercise. *
* From Rupp JC: Exercise physiology. In Roitman JL, Bibi KW, Thompson WR, editors: ACSM health fitness certification review, Philadelphia, 2001, Lippincott Williams & Wilkins; Seto C: Basic principles of exercise training. In O’Connor F, Sallis R, Wilder R, et al, editors: Sports medicine: just the facts, New York, 2005, McGraw-Hill.
Blood Volume Changes
- •
Total blood volume increases because of an increased number of red blood cells and expansion of the plasma volume. *
Blood Lipids
- •
Total cholesterol can be decreased in individuals with hypercholesterolemia.
- •
High-density lipoprotein cholesterol increases with exercise training.
- •
Low-density lipoprotein cholesterol can remain the same or decrease with regular exercise.
- •
Triglycerides can decrease in those with elevated triglycerides initially. This change is facilitated by weight loss. *
Body Composition
- •
Total body weight usually decreases with regular exercise.
- •
Fat-free weight does not normally change.
- •
Percent body fat declines. *
Biochemical Changes
- •
Stored muscle glycogen increases.
- •
The percentage of fast- and slow-twitch fibers does not change, but the cross-sectional area occupied by these fibers may change because of selective hypertrophy of either fast- or slow-twitch fibers. *
Energy System Changes
- •
Specificity of training refers to the fact that the changes that occur are specific to the muscles and energy systems that are being used.
- •
Chronic anaerobic training using the ATP–creatine phosphate system results in improved capacity and power of this system because of enhancement of enzyme activity and increases in the amount of ATP and creatine phosphate in the muscle.
- •
Anaerobic glycolysis is improved if the training program uses this system, resulting in increased stores of muscle glycogen and improved ability of enzymes in the system.
- •
Regular aerobic training improves
. It increases muscle glycogen and triglyceride stores, as well as the rate at which carbohydrates and fat are metabolized. *
ATP, Adenosine triphosphate; , maximal oxygen consumption.
Detraining
The changes induced by regular exercise training generally are lost after 4 to 8 weeks of detraining. If training is reestablished, the rate at which the training effects occur does not appear to be faster.
Overtraining
Overtraining fatigue syndrome presents as a prolonged decreased sport-specific performance, usually lasting greater than 2 weeks. It is characterized by premature fatigability, emotional and mood changes, lack of motivation, infections, and overuse injuries. Recovery is markedly longer and variable among affected athletes, sometimes taking months before the athlete returns to baseline performance ( Box 15-3 ).
- •
Sudden decline in quality of work or exercise performance
- •
Extreme fatigue
- •
Elevated resting heart rate
- •
Early onset of blood lactate accumulation
- •
Altered mood states
- •
Unexplained weight loss
- •
Insomnia
- •
Injuries related to overuse
Exercise Prescriptions
Exercise prescriptions are designed to enhance physical fitness, promote health by reducing risk factors for chronic disease, and ensure safety during exercise participation. The fundamental objective of the prescription is to bring about a change in personal health behavior to include habitual physical activity. The optimal exercise prescription for an individual is determined from an objective evaluation of that individual’s response to exercise, including observations of HR, blood pressure, rating of perceived exertion (RPE) to exercise, electrocardiogram when appropriate, and measured directly or estimated during a graded exercise test. The exercise prescription should be developed with careful consideration of the individual’s health status, medications, risk factor profile, behavioral characteristics, personal goals, and exercise preferences.
Components of an Exercise Prescription
- •
Mode is the particular form or type of exercise. The selection of mode should be based on the desired outcomes, focusing on exercises that are most likely to sustain participation and enjoyment.
- •
Intensity is the relative physiologic difficulty of the exercise. Intensity and duration of exercise interact and are inversely related.
- •
Duration or time is the length of an exercise session.
- •
Frequency refers to the number of exercise sessions per day and per week.
- •
Progression (overload) is the increase in activity during exercise training, which, over time, stimulates adaptation.
These five essential components apply when developing an exercise prescription for individuals of all ages and fitness levels. Each component of fitness (e.g., cardiorespiratory endurance, muscular strength and endurance, and flexibility) has its own specific exercise prescription associated with it. The following section reviews the ACSM guidelines for each component of fitness.
Exercise Prescription for Cardiorespiratory Endurance
Cardiorespiratory endurance is the ability to take in, deliver, and use oxygen. It is dependent on the function of the cardiorespiratory system (heart and lungs) and the cellular metabolic capacities. The degree of improvement that can be expected in cardiorespiratory fitness is directly related to the frequency, intensity, duration, and mode of exercise. can increase between 5% and 30% with training. It has become apparent recently, however, that the level of physical activity necessary to achieve the majority of health benefits is less than that needed to attain a high level of cardiorespiratory fitness.
ACSM Recommendations for Cardiorespiratory Endurance Training
Mode
- •
The best improvements in cardiorespiratory endurance occur when large muscle groups are engaged in rhythmic aerobic activity.
- •
Various activities can be incorporated into an exercise program to increase enjoyment and improve compliance.
- •
Appropriate activities include walking, jogging, cycling, rowing, stair climbing, aerobic dance (“aerobics”), water exercise, and cross-country skiing as examples.
Intensity
- •
ACSM recommendations describe minimal exercise intensity as exercise of at least moderate intensity (i.e., 40% to 60% of
that noticeably increases HR and breathing).
- •
A combination of moderate and vigorous exercise (≥60% of
that results in substantial increases in HR and breathing) is ideal for the attainment of improvements in health and fitness in most adults.
Calculating Intensity.
Because of limitations in using calculations for prescribing intensity, the most common methods of setting the intensity of exercise to improve or maintain cardiorespiratory fitness use HR and RPE.
Heart Rate Methods.
Heart rate is used as a guide to set exercise intensity because of the relatively linear relationship between HR and percentage of . It is best to measure HR max during a progressive exercise test whenever possible, because HR max declines with age. HR max can be estimated with the following equation: HR max = 220 − age. This estimation has significant variance, with a standard deviation of 10 beats/min.
Maximum Heart Rate Method.
One of the oldest methods of setting the target HR range uses a straight percentage of the HR max . Using 70% to 85% of an individual’s HR max approximates 55% to 75% of and provides the stimulus needed to improve or maintain cardiorespiratory fitness. For example, if the HR max is 180 beats/min, then the target HR (70% to 85% of HR max ) would range from 126 to 153 beats/min. (See also Chapter 27 .)
Heart Rate Reserve Method.
The HR reserve (HRR) method is also known as the Karvonen method. In this method the target range is calculated as follows: subtract standing resting HR (HR rest ) from HR max to obtain HRR. Calculate 50% and 85% of the HRR. Add each of these values to the HR rest to obtain the target range. Therefore the target range is as follows:
Low Target HR = [ ( HR max − HR rest ) × 0.50 ] + HR rest High Target HR = [ ( HR max − HR rest ) × 0.85 ] + HR rest
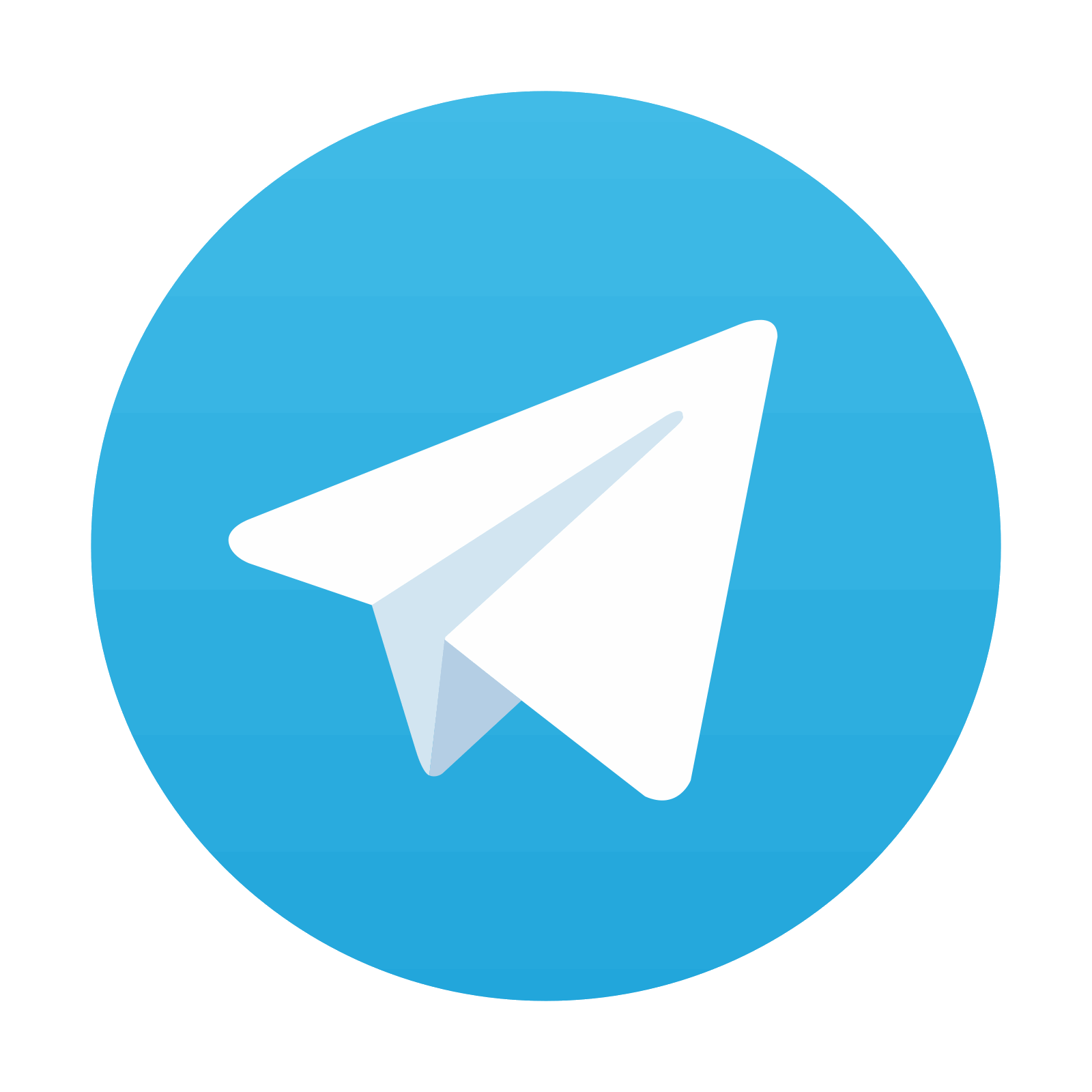
Stay updated, free articles. Join our Telegram channel
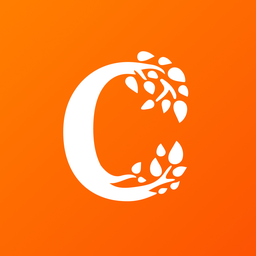
Full access? Get Clinical Tree
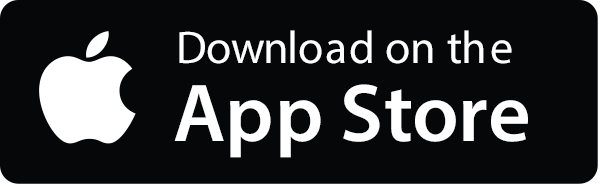
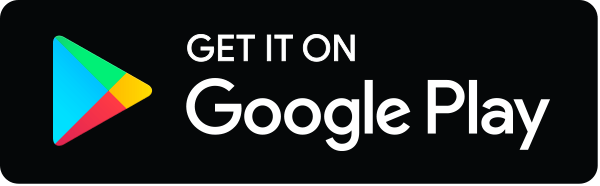