Traumatic brain injury (tbi) is classically defined as an insult to the brain caused by an external physical force with a resulting alteration in brain function. The insult can be the result of blunt trauma, penetrating, acceleration-deceleration, or blast wave injuries. The result of such an impact varies highly from patient to patient and has been correlated with the severity of injury.1 TBI is considered a leading cause of death and long-term disability worldwide and affects all age groups and genders; however, children and the elderly are often considered at a higher risk of experiencing a TBI.2 Despite the tremendous effort invested in TBI research, the ability to minimize chronic neurologic deficits in TBI patients has remained extremely limited. This is likely a result of the vast complexity of the pathophysiology of a TBI. In fact, it is necessary to continue to explore and understand the underlying mechanisms of a TBI and how these mechanisms relate to functional outcomes.
This chapter summarizes the current understanding of the pathophysiology of brain injury. The pathophysiology can be divided into primary and secondary injury. Primary injury is the immediate result of the traumatic event resulting from mechanical forces. Secondary injury is a result of the primary injury and results in a deleterious cascade of complex pathways causing secondary injury.
The chapter is divided into two sections: the pathophysiology of primary brain injury and the resultant secondary brain injury. The first section illustrates the various pathologies associated with the primary brain injury, that is, those that result from the initial physical or nonphysical impact to the brain. The second section illustrates the complexity of secondary brain injuries, as well as the pathophysiological change that occurs in the hours, days, and months after the initial neurologic insult.
A multitude of studies have been performed in an attempt to classify the various types of traumatic brain injury. The results of these studies have demonstrated that primary brain injuries fall into one of the following categories based on physical mechanism of injury: focal injuries, diffuse injuries, or a heterogeneous mixture of the two.3–7
Focal brain injuries are defined as injuries that occur in a localized area of the brain and are the result of direct mechanical forces applied to the skull. These forces can result in skull fracture, contusions, hematomas (subdural, epidural, and intraparenchymal), and hemorrhages both in the subarachnoid and intracranial spaces.
One of the most immediate effects of blunt force trauma to the skull is the potential for a resulting skull fracture. Skull fractures are classified by the location and force of the impact, as well as the size and shape of the object that made the impact to the skull. The types of fractures that can be seen with an impact to the head include closed, open, compound, depressed, basal, linear, and comminuted fractures. A closed fracture occurs when the skin that overlies the fracture is not cut or open. An open fracture occurs when the skin is cut or open and the bone is visible. A compound fracture occurs when the trauma breaks skin, bone, and dura mater, leading to the exposure of brain tissue, and typically requires surgical intervention to repair. Compound fractures also have a higher probability of infection due to the exposure of the brain to the outside environment. A depressed fracture will result in the broken skull being pushed into the brain cavity. A basal fracture is present in the base or floor of the skull. A linear fracture is a straight fracture or small cracks that are relatively simple and typically do not require treatment (Fig. 18–1). Fractures can cause bleeding, bruising, severe pain, and swelling at the site of the trauma as well as more minor symptoms. Skull fractures that occur at the base of the skull can cause further complications, as cranial nerves can often be damaged. The olfactory nerve, facial nerve, and the vestibulocochlear nerve are the most commonly injured with fractures accompanying a TBI. Treatment of skull fractures varies depending on the type of fracture and its severity.
Figure 18–1
Head CT in bone window. There is a linear skull fracture in the left occipital region (arrow). There is also an underlying epidural hematoma which is not adequately visualized in this bone window. (Reproduced with permission from Oropello JM, Mistry N, Ullman JS. Head Injury. In: Hall JB, Schmidt GA, Kress JP, eds. Principles of Critical Care, 4e New York, NY: McGraw-Hill; 2014.)

The most common cause of death following a TBI is often cited as intracranial hematomas. Compression of the brain tissue occurs as blood accumulates within the brain tissue and can result in an increase in intracranial pressure due to the closed nature of the skull. The classification of hematomas is determined by the location of blood within or outside of the meninges that surround the brain and include epidural, subdural, intracerebral, and subarachnoid hematomas (Figs. 18–2A and 18–2B). An epidural hematoma occurs between the skull and the dura mater. The middle meningeal artery is often the source of an epidural hematoma, as its anterior branch runs beneath the pterion, where the skull is relatively thin and therefore more susceptible to injury. Bleeding occurs rapidly, so immediate surgical attention is required for this type of hematoma. A subdural hematoma occurs between the dura and arachnoid mater. Subdural hematomas occur in approximately 30% of all severe TBI injuries. They are usually caused by the tearing of veins from the cortex due to the rapid acceleration/deceleration of the head during the impact. Importantly, this type of hematoma can occur acutely, subacutely, and chronically. Chronic forms may accumulate slowly and remain unnoticed for weeks. Finally, subarachnoid hemorrhages occur between the arachnoid and pia mater, and are the most common type (44% in severe TBI cases) found after TBI. A complication that can result from a subarachnoid hemorrhage is hydrocephalus due to blockade of the cerebrospinal fluid found within this meningeal layer.
Figure 18–2
(A) Acute epidural hematoma after head trauma in a 5-year-old patient. (Reproduced with permission from Pandey AS, Thompson B. Neurosurgery. In: Doherty GM, eds. CURRENT Diagnosis & Treatment: Surgery, 14e New York, NY: McGraw-Hill; 2014.) (B) CT scan showing a focal right frontoparietal intraparenchymal hematoma (arrow) associated with a subdural hematoma. There is shifting of the midline to the left due to mass effect from the hematomas (arrow). This patient ultimately required a decompressive craniectomy for elevated intracranial pressure. (Reproduced with permission from Oropello JM, Mistry N, Ullman JS. Head Injury. In: Hall JB, Schmidt GA, Kress JP, eds. Principles of Critical Care, 4e New York, NY: McGraw-Hill; 2014.)

Intracerebral hemorrhage occurs due to injury of small vessels within the brain. The impact of this type of injury is dependent on the amount of blood that leaks out of the vessel, as well as the location of the bleed. Delayed or enlargement of an intracerebral hematoma is a common occurrence, as 65% of patients with normal computed tomography (CT) scans upon presentation of a TBI were normal but over subsequent weeks developed progressive functional deficits and were rescanned to find the presence of a hematoma or an increase in the size of a hematoma.
Diffuse brain injuries are defined as injuries that occur in a larger, more generalized area of the brain and can include diffuse axonal injury, vascular injury, and cerebral concussion or contusion.
Axonal injury is the most common result of a diffuse TBI and is considered the hallmark injury of TBI, with devastating consequences. This was originally described by Strich, a pathologist, in 1956, and was later redefined by Adams as diffuse axonal injury (DAI), which is how it is universally referred to today.8,9 DAI occurs due to the sum of the rotational forces caused by rapid head acceleration/deceleration applied to the brain during the initial impact. DAI is considered a clinicopathological syndrome in which the shearing forces cause significant damage (stretching, tearing, shearing, and compression of axons and reactive swelling within the surrounding tissue), most commonly observed in white matter tracts such as the corpus callosum, internal capsule, brainstem, and the cerebral peduncles9,10 (Figs. 18–3A and 18–3B). Damage to the white matter can cause disruption of the axonal cytoskeleton and neurochemical changes, especially elevated axonal calcium levels.11–14 The results of DAI vary from loss of consciousness to coma and death. Adams et al describes a three-grade classification system for DAI. Grade I involves the grey-white matter junction; grade II involves the corpus callosum plus grade I locations; and grade III is the most severe, involving the brainstem in addition to grade I and II locations.9
Figure 18–3
(A) Diffuse axonal injury. The arrowheads indicate spheroids. Spheroids are axonal swellings associated with diffuse axonal injury. Diffuse axonal injury is most commonly associated with severe head injury; however, spheroids can also occur as a result of ischemic, infectious, and various other processes. Hematoxylin and eosin, 400×. (Reproduced with permission from Chapter 11. Neuropathology. In: Kemp WL, Burns DK, Brown TG, eds. Pathology: The Big Picture, New York, NY: McGraw-Hill; 2008.) (B) Diffuse axonal injury and diffuse vascular injury are evidenced by small hemorrhages in the corpus callosum and basal ganglia. (Reproduced with permission from Armao D, Bouldin T. Pathology of the Nervous System. In: Reisner HM, eds. Pathology: A Modern Case Study, New York, NY: McGraw-Hill; 2015.)

DAI is difficult to detect with conventional imaging techniques typically used to assess a TBI patient. However, recent advances in neuroimaging techniques may be sensitive to detect these cellular changes, particularly diffusion tensor imaging.15 In addition to imaging, recent evidence suggests that several blood biomarkers may provide additional evidence supporting that axonal injury occurs in even mild TBI. Three studies have shown that elevations in tau, an axon-enriched microtubule-associated protein, and a proteolytic fragment of axonal protein spectrin were found to be elevated in mild TBI patients.16–18
In addition to damage to the white matter of the brain, the mechanical forces of an impact cause injuries to the vasculature of the brain, resulting in vascular injury and potentially subsequent hemorrhage. Damage to the vasculature can be particularly devastating, as the brain is highly dependent on a continuous blood supply to provide nutrients to the brain, especially to support central nervous system (CNS) metabolism. CNS function has been shown to be highly coupled to neuronal activity.19 The blood–brain barrier (BBB), formed by interactions between astrocytic endfeet, endothelial cells, and a thick basement membrane, functions to maintain a constant environment for the brain by regulating the entry of substances from the vasculature. Clinical and animal research have provided substantial evidence that BBB breakdown occurs frequently following TBI and can last from several days to weeks, and in some cases, studies have shown it can persist for years after the initial injury.20,21 TBI-induced breakdown of the BBB is followed by vasogenic edema formation,22,23 increased intracranial pressure, decreased cerebral blood flow (CBF), decreased energy metabolism, and subsequent ischemia.24,25 Cytotoxic (cellular) edema due to the loss of adenosine triphosphate (ATP)–maintained ion homeostasis generally precedes vasogenic edema.26–28 TBI impairs oxygen delivery to the brain, decreasing mitochondrial function and energy production.29,30 Of these secondary processes, BBB disruption is of central importance, since it affects edema formation and determines the biochemical environment for tissue repair. There is a growing consensus that post-traumatic BBB disruption is one of the major factors that increases the severity of TBI31 and that BBB disruption may be multiphasic.32 This indicates that BBB leakage may be a target for therapeutic invention.33–35 Human and animal studies have reported varying changes in CBF following TBI, with some reporting hypoperfusion and others reporting heterogeneous changes (hypoperfusion and hyperperfusion).35–38 The disruptions in CBF and vasoreactivity (VR) may be the result of direct injury to vessels, reduction of perfusion pressure by elevated intracranial pressure, or metabolic disturbances within the neurovascular unit. Disruption of CBF and VR could lead to subsequent (secondary injury) metabolic stress, vascular dysfunction, neuronal dysfunction, hypoxic-ischemic damage, and cell death.
A cerebral cortical contusion, or bruising of the brain parenchyma due to small blood vessel leaks, is often caused by the initial impact. The contusion can occur at the site of the impact or on the opposite side of the brain, and the severity can vary highly from little to no damage to severe (Fig. 18–4). Higher severity contusions are typically correlated with the length of time a patient remains unconscious following the initial injury. Computerized tomography images can vary in appearance from a “salt and pepper” appearance to a more homogenous appearance.39 Cerebral edema can occur following a contusion to the brain and can lead to subsequent damage to the brain, potential herniation of the brain, and potentially coma. The mechanisms underlying the progressive nature of a contusion are rooted in events related to signs of hemorrhage, edema formation, inflammation, and ischemic necrosis. The initial inflammatory response typically begins in the first 24 hours postinjury within the affected area where studies have shown an invasion of polymorphonuclear leukocytes. Over the next several days microglia, macrophages, and other inflammatory cells infiltrate the area. Free radicals also contribute to the progression of the lesion, as they are released by the damaged neurons, astrocytes, and inflammatory cells, which cause increased edema formation and DNA damage. It is believed that cell death occurs through two main pathways: apoptosis and necrosis.40,41
Figure 18–4
Mechanisms of cerebral contusion. Arrows indicate point of application and direction of force; dark red areas indicate location of contusion. (A) Frontotemporal contusion consequent to frontal injury. (B) Frontotemporal contusion following occipital injury. (C) Contusion of temporal lobe because of contralateral injury. (D) Frontotemporal contusion as a result of injury to opposite temporooccipital region. (E) Diffuse mesial temporooccipital contusion caused by a blow on the vertex. (Reproduced with permission from Chapter 35. Craniocerebral Trauma. In: Ropper AH, Samuels MA, Klein JP, eds. Adams & Victor’s Principles of Neurology, 10e New York, NY: McGraw-Hill; 2014.)

While the intracranial location and severity of injury contribute to the extent of functional deficits, there are also significant contributions from physiological changes that occur during the hours to days after injury. Treating or preventing these secondary injuries is an important step in clinical management of TBI. The progression of biochemical, cellular, and molecular changes following TBI lead to ischemic/hypoxic neuronal damage, ischemic/reperfusion injury, mitochondrial dysfunction, excitotoxicity, neuronal degeneration, inflammation, BBB dysfunction, and edema formation.42,43 These changes in the cellular microenvironment contribute to the secondary injury phase when neuronal loss either permanently disables victims, decreasing their quality of life, or results in death. Unfortunately, the contribution of secondary injury to the final outcome still remains poorly understood.
Disruption in CBF can create an oxygen deficit in the brain parenchyma and result in significant cerebral ischemia and subsequent hypoxic injury with cell death.44 This secondary injury can occur along a spectrum of severity, but is typically more pronounced with severe TBI and is associated with poor outcomes.45 Ischemic injury occurs at any point during the acute phase after TBI and is due to changes in intracranial pressure (ICP) that disrupt CBF and tissue perfusion. This could be caused directly by intracranial injury or as a consequence of a general hypotensive event in a medically unstable patient. Ischemia triggers multiple local/cellular responses, including depolarization of the neuronal cell membrane and cellular swelling; oxidative damage to lipids, proteins, and nucleic acids by reactive oxygen species (ROS); cellular energetic/mitochondrial dysfunction (see later); and activation of inflammatory pathways (Fig. 18–5).
Figure 18–5
Mechanisms contributing to neuronal injury during ischemia-reperfusion. Several pathways contribute to excitotoxic neuronal injury in ischemia, with excess cytosolic Ca2+ playing a precipitating role. DAG, diacylglycerol; GluR, AMPA/kainate type of glutamate receptors; IP3, inositol trisphosphate; mGluR, metabotropic glutamate receptor; NMDA-R, N-methyl-D-aspartate receptor; O2−, superoxide radical; PIP2, phosphatidylinositol 4,5-bisphosphate; PKC, protein kinase C; PL, phospholipids, PL phospholipase; VSCC, voltage-sensitive Ca2+ channel; COX, cyclooxygenase; LOX, lipoxygenase; NCX, NA+/Ca2+ exchanger; mtPTP, mitochondrial permeability transition pore. (Reproduced from Dugan LL, Kim-Han JS. Hypoxic-ischemic brain injury and oxidative stress. In: Siegel GS, et al, eds. Basic neurochemistry: molecular, cellular, and medical aspects, 7th ed. Burlington, MA: Elsevier Academic Press; 2006: 564. Copyright © 2006, American Society for Neurochemistry. All rights reserved.)

Restoration of CBF may also lead to cerebral reperfusion injury, which carries the risk of cerebral edema and intracranial bleeding and which results in damage to tissue not injured with the initial insult. Reperfusion injury is a complex process that can occur in different organs. As is the case with ischemia, ROS (specifically peroxynitrite) play a significant role in reperfusion injury with damage occurring at the cellular and molecular level that ultimately activates apoptotic pathways. Tissue injury is also thought to be partly mediated through activation of the immune system, the complement system, and platelets and coagulation cascade components.46 Controlling CBF is understood to be a critical step in minimizing secondary ischemic and reperfusion injuries; therefore, ICP monitoring and therapeutic interventions aimed at maintaining ICP in severe TBI have been the cornerstones in acute TBI care.
Mitochondria are considered essential organelles for cell survival, as they generate the majority of the cell’s energy production by oxidative phosphorylation. Mitochondria are sensitive to changes in the physiological state of a cell, and their dysfunction has been implicated in numerous neuropathological processes, including TBI. Increasing evidence suggests that TBI leads to both direct mechanical damage and functional disturbances in mitochondria and plays a key role in contributing to apoptotic and necrotic cell death. As the brain is a highly aerobic, energy-demanding tissue, an imbalance in energy demand for repair of cellular damage and concomitant decrease in energy generation caused by mitochondrial dysfunction leads to increased cellular damage. Excitotoxicity, production of ROS, Ca2+ overload, apoptosis-inducing factor, and caspases are some of the primary candidates that induce mitochondrial damage following TBI.29,30,47 During injury, the surge in ROS facilitates a vicious cycle that accelerates mitochondrial damage, excitotoxicity, lipid peroxidation, and inflammation.48 Mitochondrial membrane potential is a key indicator of damage to the mitochondria and may be linked to early-stage apoptosis. Thus, mitochondrial-targeting strategies in TBI have been increasingly studied, as their maintenance could potentially preserve brain function.49,50
Data suggest that oxidative stress contributes to the overall pathology following TBI, in part through the modulation of autophagy.51 Autophagy can act as a defense mechanism against oxidative stress by degrading oxidized proteins and damaged mitochondria (mitophagy)52,53 (Fig. 18–6). Oxidative damage to mitochondrial membrane proteins causes proteins to misfold, aggregate, and form nonspecific aqueous pores, whose opening leads to the mitochondrial permeability transition (MPT); MPT is thought to be an inner membrane permeabilization which may precede eventual cell apoptosis and death.52,54,55 In excess, the MPT leads to necrosis from ATP depletion after uncoupling of oxidative phosphorylation or apoptosis due to caspase activation by cytochrome-c released after MPT-induced mitochondrial swelling.56 However, with less severe stress, MPT pore formation appears to promote mitophagy.55 Following oxidative stress induced by TBI, the opening of the MPT has been implemented as the “critical switch” which determines cell death or survival.54 The development of novel treatment options to prevent the evolution of the MPT from the level of mitophagy induction to that of apoptosis and necrosis following TBI could lead to improved pathology.
Figure 18–6
Autophagy is a process in which the cell uses lysosomes to dispose of excess or nonfunctioning organelles or membranes. Membrane that appears to emerge from the smooth endoplasmic reticulum encloses the organelles to be destroyed, forming an autophagosome that then fuses with a lysosome for digestion of the contents. In this picture taken by transmission electron microscopy the two autophagosomes at the upper left contain portions of rough endoplasmic reticulum (RER) more electron dense than the neighboring normal RER, and one near the center contains what may be mitochondrial membranes plus RER. Also shown is a vesicle with features of a residual body (30,000X). (Reproduced with permission from The Cytoplasm. In: Mescher AL, eds. Junqueira’s Basic Histology, 14e New York, NY: McGraw-Hill; 2016.)

Substantial evidence points to excitotoxicity as a fundamental mechanism involved in neuronal degeneration and neuronal injury following brain trauma.57 Excitotoxicity is defined as cell death resulting from the toxic actions of excitatory amino acids, especially glutamate.57 Following trauma, the excessive activation of glutamate receptors results in excitotoxicity that leads to neuronal dysfunction and death, likely through an oxidative stress mechanism.57 One of the major ionotropic receptors activated by glutamate following TBI is N-methyl-D-aspartic acid (NMDA), a ligand-gated channel that is favorably permeable to Ca2+.57 NMDA receptor expression is highly characterized in neurons and more recently has been shown to be expressed in astrocytes.58,59 Continuous activation of NMDA receptors results in increased intracellular calcium and catabolic enzyme activity, triggering oxidative stress cascades that cause membrane degradation and BBB compromise, resulting in apoptosis and necrosis.58,59 Downstream effects of NMDA activation include mitochondrial membrane depolarization resulting in MPT, caspase activation, production of toxic oxygen and nitrogen-free radicals, and cellular toxicity.55 Autophagy is activated and plays a role in neuronal outcome in response to NMDA-induced toxicity.58 Excitotoxicity via overstimulation of NMDA receptors is a hallmark secondary event following brain trauma, but the role of autophagy in this process is unclear.58
The blood–brain barrier, also known as the neurovascular unit, has the primary function to prevent movement of unwanted substances from the body into the central nervous system. The BBB is composed of endothelial cells, glial cells, microglia, pericytes, and smooth muscle cells of the vasculature.60 Tight junctions restrict movement of substances through the BBB, with transport occurring through either active or passive transport. TBI-induced vasogenic edema arises from transvascular leakage caused by mechanical failure of endothelial tight junctions (TJs) of the BBB. TJs consist of integral proteins (claudin and occludin) and accessory proteins (zonula occludens, z01 and z02) that are necessary for structural support of the BBB. The cerebral endothelial cells and their TJs are surrounded by and functionally influenced by astrocytic perivascular endfeet.
Following an injury to the brain, the brain parenchyma is typically damaged, which leads to cerebral microvessels becoming damaged and results in the dysfunctioning of the BBB. Studies using magnetic resonance imaging (MRI) and IgG or Evans blue extravasation have shown that the BBB can initially open as early 30 minutes to a day after a TBI and can remain open for up to 30 days postinjury.20,61,62 Interestingly, the opening of the BBB occurs in a biphasic manner, peaking between 4 and 6 hours and again 3 days postinjury.63 The initial disruption of the BBB initiates a coagulation cascade that can lead to ischemia in the surrounding impact area and the release of blood factors such as thrombin, fibrinogen, and albumin into the brain tissue and initiate a pro-inflammatory response.64,65 Other studies have found that claudin-5 may play an important role in regulating transport, as it is highly expressed on the microvasculature endothelium within the brain and its expression changes postinjury.66 The increase in claudin-5 protein expression has been noted to occur 1 to 2 weeks after injury, and can remain elevated up to 4 to 8 weeks.66,67 One study evaluated the spatiotemporal evolution of BBB using an MRI technique called Ktrans and demonstrated that the predominate location of BBB disruption occurs in the superficial layers of the cortex and diminished as a function of distance from the impact site.68 The data were compared to edema formation and cerebral blood flow changes in the same region and suggested that each component may be related to multiple components of the impact itself, with cell death primarily found in the tissue of the superficial impact zone and progressively declines as a function of distance. However, CBF change and edema were found to occupy a much larger area around the impact zone and suggests this area is also affected.68
Cerebral edema is defined as an abnormal accumulation of fluid within the brain parenchyma, which results in swelling of the brain. The swelling leads to increased ICP, which can lead to brainstem compression, compromised circulation, and death if untreated (Fig. 18–7). Cerebral edema is thought to be a leading cause of death in severe TBI patients, with some studies reporting up to half of all the mortality in TBI occurring due to edema formation.69
Figure 18–7
Cerebral edema due to subarachnoid hemorrhage versus the normal brain. The brain on the right side had a subarachnoid hemorrhage, which caused cerebral edema. Note the flattened gyri and narrowed sulci. In comparison, the gyral surfaces of the normal brain on the left are gently rounded, and the intervening sulci are well preserved. (Reproduced with permission from Chapter 11. Neuropathology. In: Kemp WL, Burns DK, Brown TG, eds. Pathology: The Big Picture New York, NY: McGraw-Hill; 2008.)

Four generalized forms of edema may occur following a TBI: vasogenic, cytotoxic, osmotic, and interstitial cerebral edemas. Vasogenic cerebral edema occurs when the blood–brain barrier is disrupted following the injury, allowing water to move from the vasculature to the extracellular space within the brain, leading to increased ICP. Cytotoxic edema is defined as accumulation of fluid within the intracellular compartment of a cell following injury. Studies have demonstrated that water accumulation into the intracellular compartment is associated with the failure of the ATP-dependent NA+/K+ pumps following energy failure. Failure of these pumps then increases the cellular ionic content and increases cell osmolality, which results in an influx of water into the cell, thereby altering the intracellular concentration of metabolites. This form of edema does not change the overall brain volume, and therefore is not associated with a rise in ICP.
TBI can cause the breakdown of the BBB, which results in vasogenic edema formation,22,23 increased ICP, decreased cerebral blood flow, and subsequent ischemia.24,25,70 There has long been a debate as to which type of edema dominates following a TBI, although it was generally believed that TBI primarily induces vasogenic edema due to the opening of the BBB.71,72 However, both forms have been found to exist postinjury26–28,62,73 and have been shown to be the highest between 24 and 72 hours after the initial injury. More recent newer studies have shown that cerebral edema occurs in a biphasic manner and begins within hours of the TBI.26,74–76
Inhibition of glial mitochondrial metabolism increases astrocyte swelling and necrotic cell death, which has been implicated as a primary cause of cytotoxic brain edema.77–80 TBI impairs oxygen delivery to the brain, decreasing mitochondrial function and energy production.81–84 Central to the regulation of water homeostasis are aquaporins, a family of membrane water channels that provide the major route for bidirectional water movement by simple diffusion along osmotic and hydrostatic gradients in a variety of cell types.85–87 Aquaporin 1, 4, and 9 have been found to be expressed within the brain.88 Aquaporin 4 (AQ4) is the primary water channel expressed within astrocytic endfeet surrounding the brain vasculature.85,89–91 It is presumed to play a key role in edema formation, especially during a state of inhibited energy production, and many studies have shown that AQ4 is altered following TBI.92–95 AQP4 is upregulated by ischemia, traumatic brain injury, and a diverse array of other CNS pathologies.91,96 However, studies in AQ4-null mice have suggested a role in preventing cytotoxic edema formation during both ischemic and toxic injuries.1 Protein kinase C (PKC) is a cytoplasmic serine threonine kinase that has been shown to phosphorylate AQP4 when expressed in Xenopus laevis oocytes and that water permeability of AQP4 is decreased by PKC activation.97 Activation of PKC has been reported to decrease AQ4 expression and subsequent edema formation and may provide a mechanism for P2Y1R-mediated neuroprotection. The physiological significance of these findings has not yet been revealed.
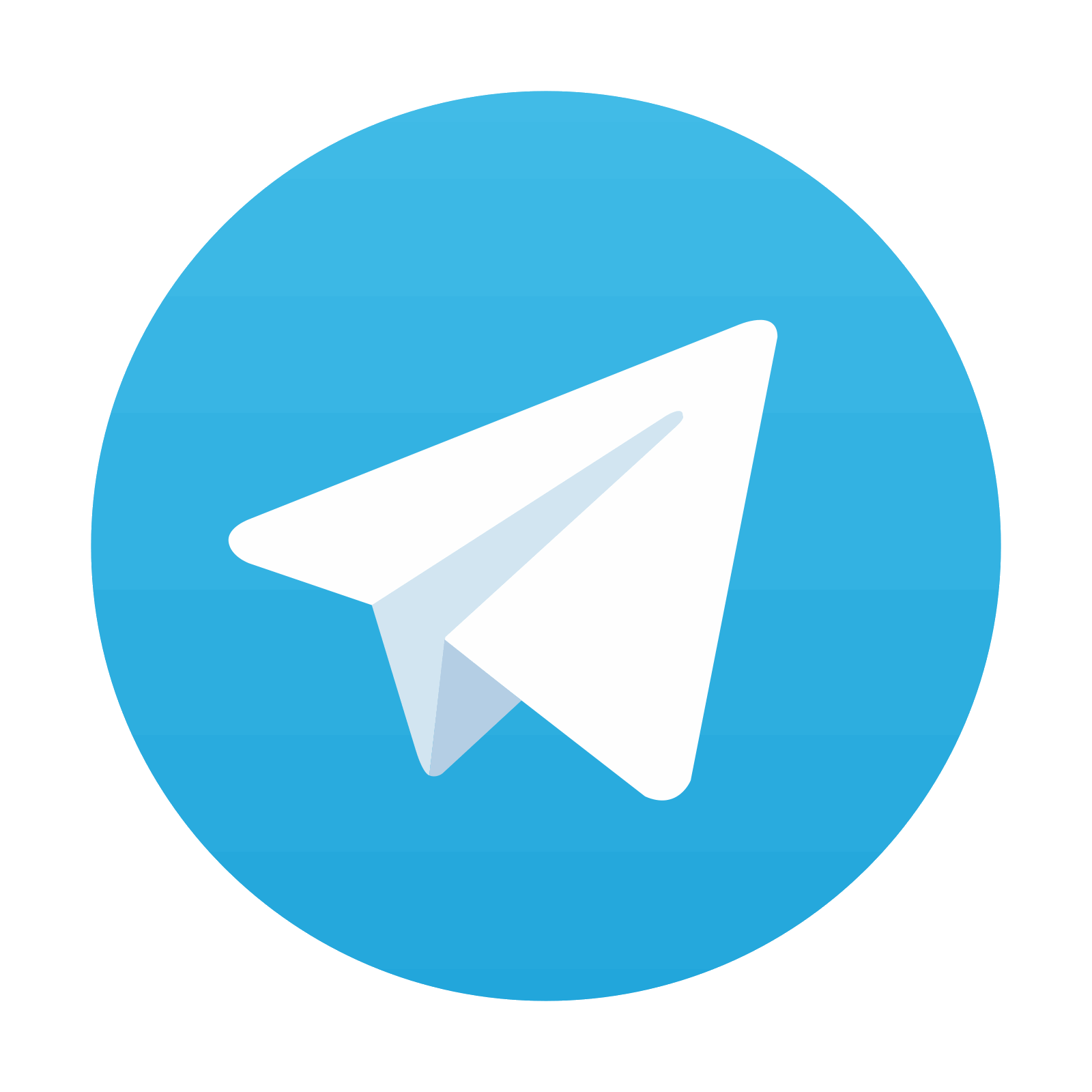
Stay updated, free articles. Join our Telegram channel
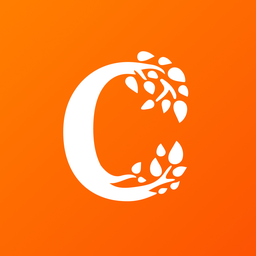
Full access? Get Clinical Tree
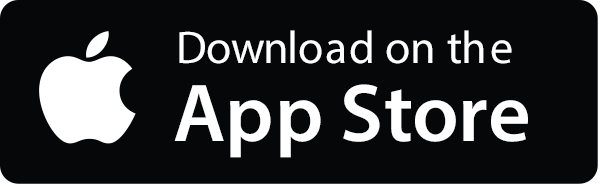
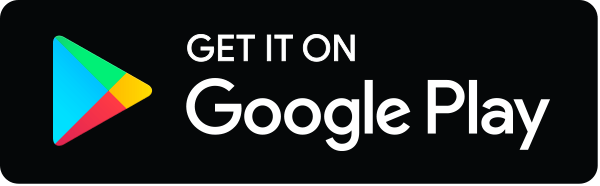