Mild traumatic brain injury, especially sport-related concussion, is common among young persons. Consequences of transient pathophysiologic dysfunction must be considered in the context of a developing or immature brain, as must the potential for an accumulation of damage with repeated exposure. This review summarizes the underlying neurometabolic cascade of concussion, with emphasis on the young brain in terms of acute pathophysiology, vulnerability, alterations in plasticity and activation, axonal injury, and cumulative risk from chronic, repetitive damage, and discusses their implications in the context of clinical care for the concussed youth, highlighting areas for future investigation.
Mild traumatic brain injury (TBI) or concussion is estimated to occur in millions of persons annually in the United States alone. The peak ages for these injuries are in adolescence and young adulthood, and sport-related concussions are particularly common among young persons. Although generally these injuries are self-limited, the consequences of even transient pathophysiologic dysfunction must be carefully considered in the context of a developing or immature brain, as must the potential for an accumulation of damage with repeated exposure over a long period. This article reviews the underlying neurometabolic cascade of concussion, with emphasis on the young brain in terms of acute pathophysiology, vulnerability, alterations in plasticity, axonal injury, and risk for chronic cumulative deficits.
Acute metabolic cascade
Glutamate Release and Ionic Disequilibrium
The postconcussive metabolic cascade has been well studied and characterized, both in animal models and in humans. As a result of mechanical trauma, neuronal cell membranes and axons undergo disruptive stretching, leading to temporary ionic disequilibrium. As a result, levels of extracellular potassium increase drastically, and indiscriminate glutamate release occurs. Glutamate release activates N -methyl- d -aspartate receptors, which leads to accumulation of intracellular calcium, causing mitochondrial respiration dysfunction, protease activation, and often initiating apoptosis. Increased glutamate levels were also found to be significantly correlated with derangements in lactate, potassium, brain tissue pH, and brain tissue CO 2 levels in human studies. In addition, sodium channel upregulation, fueled by adenosine triphosphatase proteins depending on glucose for energy, is observed after axonal stretch injuries.
Energy Crisis and Mitochondrial Dysfunction
In combination, the cellular response to the ionic shifts and the downstream effects of the neurotransmitter release mentioned earlier lead to an acute energy crisis. This crisis occurs when, to restore ionic equilibrium, adenosine triphosphate (ATP)-dependent sodium-potassium ion transporter pump activity increases, which augments local cerebral glucose demand. Further metabolic demand is incurred by ATP-dependent sodium channel upregulation. This demand occurs in the face of mitochondrial dysfunction, leading cells to primarily use glycolytic pathways instead of aerobic metabolism for energy, and causing extracellular lactate accumulation as a byproduct. This acidosis, caused by hyperglycolysis, has been shown to worsen membrane permeability, ionic disequilibrium, and cerebral edema.
Some evidence shows that the lactate produced by this process may eventually be used as a source of energy by the neurons once mitochondrial oxidative respiration normalizes; 1 study showed that in moderate to severe TBI the incidence of abnormally high levels of lactate uptake was seen in 28% of patients. The same study showed that patients showing a higher rate of brain lactate uptake relative to arterial lactate levels tended to have more favorable outcomes compared with others with lower relative lactate uptake.
Alterations in Cerebral Blood Flow
Cerebral blood flow (CBF) changes after severe TBI have also been well studied. A triphasic response to severe TBI has been characterized, specifically showing hypoperfusion on postinjury day 0, hyperperfusion on postinjury days 1 to 3, and vasospasm on postinjury days 4 to 15. Regarding mild TBI, some studies have shown that CBF decreases immediately after the insult, and the amount of time it remains decreased seems to depend on the severity of the injury. However, other studies show no significant differences in CBF after mild TBI in patients more than 30 years of age. In pediatric studies, CBF has been seen to increase during the first day after mild TBI, followed by decreased CBF for many days after. Data comparing CBF in pediatric patients with TBI have shown impaired autoregulation in 42% of moderate and severe and 17% of mild injuries. In severe TBI, impaired autoregulation was associated with worsened long-term outcomes.
Vulnerability to second injury and second impact syndrome
Postconcussive physiologic changes have been shown to increase the vulnerability of the brain to further injury, particularly in cases in which a second concussive injury is sustained within days of the first. This phenomenon can lead to more severe and permanent deficits. Numerous studies, both in animal models and in humans, support the concept of postconcussive vulnerability, prompting the development of many sets of return-to-play guidelines. Research has shown that having a history of concussion increases an individual’s probability of having a future concussion as well as prolonging the duration of significantly abnormal cognitive functioning. These data support increased awareness of postconcussive vulnerability and suggest adherence to return-to-play guidelines may be helpful.
In addition, an extreme entity termed second impact syndrome has been postulated, in which an individual experiencing a second mild TBI within days of the first can experience diffuse cerebral swelling and catastrophic deterioration. Second impact syndrome remains somewhat controversial in definition and explanation, with most of the literature addressing its existence being case reports. The pathophysiology of second impact syndrome is believed to result from the combined effects of increased intracranial blood volume (secondary to loss of CBF autoregulation) and posttraumatic catecholamine release, leading to catastrophic cerebral edema, generally in the absence of major hematomas or other space-occupying lesions. McCrory and Berkovic proposed diagnostic criteria in 1998 to facilitate more consistent analysis of potential cases of second impact syndrome, as well as to diminish the bias involved in case reporting. After applying these criteria, many of the previously reported cases of second impact syndrome were found to fall short of diagnosis. Nonetheless, there is recognition of a syndrome of malignant cerebral edema after mild TBI, and multiple reviews and case series of second impact syndrome have been published. A similar syndrome has been described in individuals harboring a calcium channel mutation (CACNA1A) associated with familial hemiplegic migraine.
Experimental
Numerous basic science studies have investigated the consequences of sustaining 2 closely timed brain injuries compared with a single insult, characterizing the effects on brain damage and cognitive deficits. As described earlier, neurons undergo significant changes in metabolism, blood flow, and energy usage after a single TBI. These changes in cellular function contribute to transient dysfunction after injury, but in mild TBI, cellular recovery eventually occurs, typically with minimal anatomic changes/damage. Metabolic derangements in N -acetylaspartate°(NAA), ATP, and ATP/adenosine diphosphate ratios after concussive brain injuries in rats have been studied. Results showed that 2 concussive injuries separated by 3 days showed derangements significantly worse than either a single injury or 2 injuries with 5 days separation. A more recent study by Vagnozzi and colleagues, who measured other biochemical markers of mitochondrial dysfunction in rats sustaining 2 mild TBIs, showed comparable findings. Another notable result was that a second impact at 5 days after the first showed metabolic changes equivalent to the first impact but not more severe. Sustained oxidative and nitrosative stresses have also been shown to follow a similar pattern, with peak derangements noted with a second impact occurring 3 days after the first.
These results corroborated those of an earlier study from 2005 by Longhi and colleagues, who compared mice with 1 concussive event or 2 concussive events 1, 3, 5, or 7 days apart with control mice that underwent sham injuries. This study showed significant cognitive deficits in mice concussed twice at intervals of 3 and 5 days but not in those concussed only once, or concussed twice but at 1-day or 7-day intervals. This finding supports the notion of a defined interval after an initial insult during which there is increased propensity for greater deficits should a second trauma occur. This study also showed a significantly increased degree of traumatic axonal injury mice sustaining a second injury 3 days after the first injury when compared with mice with only a single concussion.
Other studies have focused on histologic and cognitive changes after mild TBI. It has been shown that experimental closed head injury in juvenile rats can cause cognitive deficits in the absence of gross pathology or high mortality. However, a second injury performed 1 day after the first caused increased memory impairment as well as neuropathologic changes of increased axonal injury and astrocytic reactivity. This study is also the only experimental study of repeated mild TBI conducted in immature animals, thus targeting the age group at highest risk for such injuries in humans. Another study showed impairment in the Morris water maze task after 3 sequential concussive head traumas causing transient loss of consciousness, but these injuries also caused contracoup neuropathologic changes compared with nonconcussed controls.
Human Studies
Magnetic resonance spectroscopy (MRS) has been used to assess cellular changes after sustaining TBI. Vagnozzi and colleagues, using voxels in the white matter of the bilateral frontal lobes immediately adjacent to the cortical-subcortical junction, assessed the ratio of NAA to creatine (Cr) specifically in athletes after concussion. Concussed athletes showed a decrease (−18.5%) in the NAA/Cr ratio on postinjury day 3 compared with age-matched, nonconcussed controls (1.80 ± 0.04 vs 2.15 ± 0.1; P <.001), with return to normal values by 30 days after concussion. A second concussive event prolonged the time of NAA/Cr normalization by 15 days, with NAA/Cr values remaining significantly less than normal (1.82 ± 0.1) at 30 days, and return to normalcy noted only at 45 days (2.07 ± 0.1).
Data described in these studies, coupled with the lack of consensus regarding management of concussion based on clinical evaluation alone, support supplementing clinical assessment by using biomarkers. This strategy would add more objectivity and allow for better-defined return-to-play recommendations. The necessity of including such data in gauging severity of TBI and recovery stems from showing that an absence of clinical signs or symptoms does not always coincide with completed brain metabolic recovery. Conversely, it is still unclear whether metabolic alterations in the absence of measurable clinical impairment indicate an underlying vulnerable state. A potentially valuable approach might be to identify clinical measures that closely reflect the underlying metabolic state in a subset of athletes, because it is currently impractical to propose routine use of MRS for all concussions. Ideally, the approach to management of a concussed individual would then involve monitoring of clinical status in all and metabolic biomarkers in a selected subset of athletes.
Vulnerability to second injury and second impact syndrome
Postconcussive physiologic changes have been shown to increase the vulnerability of the brain to further injury, particularly in cases in which a second concussive injury is sustained within days of the first. This phenomenon can lead to more severe and permanent deficits. Numerous studies, both in animal models and in humans, support the concept of postconcussive vulnerability, prompting the development of many sets of return-to-play guidelines. Research has shown that having a history of concussion increases an individual’s probability of having a future concussion as well as prolonging the duration of significantly abnormal cognitive functioning. These data support increased awareness of postconcussive vulnerability and suggest adherence to return-to-play guidelines may be helpful.
In addition, an extreme entity termed second impact syndrome has been postulated, in which an individual experiencing a second mild TBI within days of the first can experience diffuse cerebral swelling and catastrophic deterioration. Second impact syndrome remains somewhat controversial in definition and explanation, with most of the literature addressing its existence being case reports. The pathophysiology of second impact syndrome is believed to result from the combined effects of increased intracranial blood volume (secondary to loss of CBF autoregulation) and posttraumatic catecholamine release, leading to catastrophic cerebral edema, generally in the absence of major hematomas or other space-occupying lesions. McCrory and Berkovic proposed diagnostic criteria in 1998 to facilitate more consistent analysis of potential cases of second impact syndrome, as well as to diminish the bias involved in case reporting. After applying these criteria, many of the previously reported cases of second impact syndrome were found to fall short of diagnosis. Nonetheless, there is recognition of a syndrome of malignant cerebral edema after mild TBI, and multiple reviews and case series of second impact syndrome have been published. A similar syndrome has been described in individuals harboring a calcium channel mutation (CACNA1A) associated with familial hemiplegic migraine.
Experimental
Numerous basic science studies have investigated the consequences of sustaining 2 closely timed brain injuries compared with a single insult, characterizing the effects on brain damage and cognitive deficits. As described earlier, neurons undergo significant changes in metabolism, blood flow, and energy usage after a single TBI. These changes in cellular function contribute to transient dysfunction after injury, but in mild TBI, cellular recovery eventually occurs, typically with minimal anatomic changes/damage. Metabolic derangements in N -acetylaspartate°(NAA), ATP, and ATP/adenosine diphosphate ratios after concussive brain injuries in rats have been studied. Results showed that 2 concussive injuries separated by 3 days showed derangements significantly worse than either a single injury or 2 injuries with 5 days separation. A more recent study by Vagnozzi and colleagues, who measured other biochemical markers of mitochondrial dysfunction in rats sustaining 2 mild TBIs, showed comparable findings. Another notable result was that a second impact at 5 days after the first showed metabolic changes equivalent to the first impact but not more severe. Sustained oxidative and nitrosative stresses have also been shown to follow a similar pattern, with peak derangements noted with a second impact occurring 3 days after the first.
These results corroborated those of an earlier study from 2005 by Longhi and colleagues, who compared mice with 1 concussive event or 2 concussive events 1, 3, 5, or 7 days apart with control mice that underwent sham injuries. This study showed significant cognitive deficits in mice concussed twice at intervals of 3 and 5 days but not in those concussed only once, or concussed twice but at 1-day or 7-day intervals. This finding supports the notion of a defined interval after an initial insult during which there is increased propensity for greater deficits should a second trauma occur. This study also showed a significantly increased degree of traumatic axonal injury mice sustaining a second injury 3 days after the first injury when compared with mice with only a single concussion.
Other studies have focused on histologic and cognitive changes after mild TBI. It has been shown that experimental closed head injury in juvenile rats can cause cognitive deficits in the absence of gross pathology or high mortality. However, a second injury performed 1 day after the first caused increased memory impairment as well as neuropathologic changes of increased axonal injury and astrocytic reactivity. This study is also the only experimental study of repeated mild TBI conducted in immature animals, thus targeting the age group at highest risk for such injuries in humans. Another study showed impairment in the Morris water maze task after 3 sequential concussive head traumas causing transient loss of consciousness, but these injuries also caused contracoup neuropathologic changes compared with nonconcussed controls.
Human Studies
Magnetic resonance spectroscopy (MRS) has been used to assess cellular changes after sustaining TBI. Vagnozzi and colleagues, using voxels in the white matter of the bilateral frontal lobes immediately adjacent to the cortical-subcortical junction, assessed the ratio of NAA to creatine (Cr) specifically in athletes after concussion. Concussed athletes showed a decrease (−18.5%) in the NAA/Cr ratio on postinjury day 3 compared with age-matched, nonconcussed controls (1.80 ± 0.04 vs 2.15 ± 0.1; P <.001), with return to normal values by 30 days after concussion. A second concussive event prolonged the time of NAA/Cr normalization by 15 days, with NAA/Cr values remaining significantly less than normal (1.82 ± 0.1) at 30 days, and return to normalcy noted only at 45 days (2.07 ± 0.1).
Data described in these studies, coupled with the lack of consensus regarding management of concussion based on clinical evaluation alone, support supplementing clinical assessment by using biomarkers. This strategy would add more objectivity and allow for better-defined return-to-play recommendations. The necessity of including such data in gauging severity of TBI and recovery stems from showing that an absence of clinical signs or symptoms does not always coincide with completed brain metabolic recovery. Conversely, it is still unclear whether metabolic alterations in the absence of measurable clinical impairment indicate an underlying vulnerable state. A potentially valuable approach might be to identify clinical measures that closely reflect the underlying metabolic state in a subset of athletes, because it is currently impractical to propose routine use of MRS for all concussions. Ideally, the approach to management of a concussed individual would then involve monitoring of clinical status in all and metabolic biomarkers in a selected subset of athletes.
Impaired/abnormal activation after TBI
Experimental
Experimental brain injury models have indicated that brain activation is altered for several weeks after TBI. Changes in excitability and circuit function have not only been observed in more severe brain injury but also after mild TBI. Anatomic changes after trauma along with subtle changes in neuronal properties influence neuronal excitability. These postinjury changes in brain activation have an array of consequences ranging from alterations in synaptic plasticity, axonal sprouting and excitatory-inhibitory imbalance. These underlying physiologic changes may then manifest phenotypically as cognitive impairments or increased risk of seizures/epilepsy.
After a brain injury, metabolic alterations occur that result in subacute hypometabolism. The duration of this metabolic disturbance is believed to be associated with the severity of the injury. These metabolic changes are likely to influence brain activation. Changes in excitability also have an effect on calcium regulation and thus on molecular markers of plasticity such as neurotrophins. Animal research indicates that neurotrophin expression, such as brain-derived neurotrophic factor (BDNF), is regulated by neural activity. In turn, alterations in BDNF also influence brain activation given its role on synaptic facilitation and neurotransmitter release enhancement. For example, BDNF levels normally increase in response to exercise ; however, this is not observed if exercise occurs during the first days after a fluid-percussion injury (FPI). Among these lines, cortical stimulation to the brain after FPI has been observed to elicit a metabolic response that may act as a secondary injury by increasing cortical degeneration. Metabolic and ionic disturbances also affect function of the N -methyl- d -aspartate receptor (NMDAR), which is a key molecular player in neuronal activation and allows for long-term potentiation (LTP), a cellular correlate of learning. As a glutamate receptor, the NMDAR plays a major role in neuronal excitability. The response to injury, such as the period of metabolic depression, differs between adult and developing animals.
Changes in brain activation can also have an effect on the regulation of the hypothalamic pituitary adrenal (HPA) axis. Efficient HPA regulation is necessary for neuronal vitality and function, thus its dysregulation can have profound effects on synaptic plasticity as well as cognitive and affective well-being. Glucocorticoid release is regulated by the hypothalamus, a region receiving multiple suprahypothalamic inputs with notable projections from the limbic system. Alterations in function and connectivity are likely to exert a substantial influence on HPA regulation. Glucocorticoid receptors are widespread throughout the brain and are predominantly expressed within the hippocampus. Excitatory changes within the hippocampus thus have an effect on glucorticoid negative feedback. Recent findings from adult rodent studies indicate that there is a hyperresponsiveness to stress after a mild FPI. This hyperresponsiveness was observed during the first postinjury weeks. These increases indicate hypothalamic disruptions in neuronal circuitry. A heightening of the stress response may be particularly concerning in the developing brain. Neonatal or pubertal stress has a pronounced effect on adult behavior and brain plasticity. Along these lines, corticotropin-releasing hormone mRNA has been shown to increase after TBI. In addition to the heightened stress response, animal studies, using different injury models, have indicated other TBI-induced changes in HPA regulation.
Human Studies
As shown in animal models of injury, neurochemical and metabolic disruptions are also observed in humans. Metabolic disruptions after experimental TBI extend over a period of days and normalize after approximately 10 days, whereas changes after moderate to severe human TBI may last weeks or even months. In addition, the consequences stemming from a TBI include white matter damage and changes in activation patterns. These alterations are likely to contribute to the long-term cognitive impairments that are observed after TBI. These impairments range from acute memory problems to difficulty with higher executive functions such as strategy switching and planning. A functional magnetic resonance imaging (MRI) study of individuals with mild or moderate brain injury found neural activity alterations a year after injury. In particular, disruptions in catecholaminergic circuitry, which is associated with working memory functions, were observed. Alterations in activation after mild brain injury are also associated with axonal damage, but this is generally not apparent with computed tomography. Subtle white matter abnormalities are observed in patients with persistent postconcussive symptoms after suffering from a mild TBI using diffusion tensor imaging (DTI) (see later discussion).
As indicated earlier, changes in activation patterns are likely to have an effect on glucocorticoid regulation. Acute activation of the HPA axis occurs initially after brain injury as a protective response that modulates the immune/inflammatory response and increases metabolic substrate availability. Clinical studies have shown increases in cortisol acutely after brain trauma and these increases are correlated with the duration of coma and recovery after head trauma. As for the subacute period, studies suggest that plasma cortisol levels are dependent on the injury severity. Cortisol tends to increase after mild to moderate brain injury in the early postinjury period, whereas it is depressed after a severe injury. Although structural pituitary damage may be dependent on the severity of the injury, pituitary abnormalities have been reported in mild to moderate injuries. MRI studies indicate that 37% of adult patients with mild TBI, with a Glasgow coma score ranging from 13 to 15, had structural pituitary abnormalities. A higher rate of pituitary abnormalities was found in a pediatric study looking at functional measures. Basal cortisol levels were significantly reduced in this population. It still remains to be answered if there is an age-dependent vulnerability of the HPA axis.
Altered plasticity and development
Experimental
Brain injury leads to alterations in molecular substrates of synaptic plasticity. Of particular interest are the effects on proteins such as BDNF and the NMDAR, which are strongly linked with synaptic strengthening and play a significant role in experience-dependent plasticity. Because the young brain is undergoing developmental changes, injury-induced alterations of molecular markers of plasticity may not only affect injury outcome, as it has been shown in adults, but also deviate developmental processes from their normal trajectory.
It is during the developmental period that the brain is more susceptible to environmental influences. This finding has been shown in enriched environment (EE) studies. These studies indicate that structural changes are more likely to occur in a developing rat as a response to environmental stimulation when compared with an adult rat. However, when a developing rat is exposed to an EE after a FPI, the beneficial effects that indicate neuroplasticity, such as cortical thickening, expanded dendritic arborization, and cognitive enhancement, are not observed. The cognitive benefit that is usually found after EE exposure is found only if the period of enrichment is delayed after the injury. This failure to enrich after developmental TBI is observed despite no significant cell death. This finding shows that mechanisms driving neuroplasticity are affected after TBI and may lead to the failure to appropriately respond to environmental stimulation. In accordance with this idea, changes in NMDAR composition have been observed after an FPI in young rats. In addition, alterations in molecules that facilitate synaptic plasticity, such as BDNF, are likely contributors to the post-TBI failure of EE.
Adult TBI studies have shown that expression of BDNF is acutely altered in different models of TBI. BDNF mRNA is dynamically regulated during postnatal development in various central nervous system structures and its levels are high and unstable compared with the mature brain. Therefore, it is plausible that the effects of TBI on BDNF are age dependent. Levels of BDNF were observed to be increased after an FPI in developing rats when compared with adult FPI. These increases in BDNF were most pronounced in areas remote from the injury site and may indicate synaptic remodeling. Reorganization after brain injury has been proposed as a mechanism to facilitate recovery and has been observed in animals after brain injury.
A substantial increase in neuronal connectivity occurs during the postnatal period, as a response to functional and environmental demands. During this period, the organization of fiber projections takes place through regionally selective outgrowth or selective elimination of projections. These ongoing maturational processes emphasize the significance of TBI studies in this age range. These studies suggest that after TBI, future developmental plasticity may be compromised, in that neuroplasticity shifts from responding to experience-dependent adaptation to that of restoration/compensation to a preinjury status.
Human Studies
Data obtained from human TBI studies indicates that the capacity of the brain to compensate for injury is largely dependent on the state of cerebral maturation. For example, children younger than 4 years have a worse outcome compared with older children. Paradoxically, although children may be more behaviorally resilient to injury, cognitive deficits may not be noticeable until the child is exposed to a more demanding environment. Thus, deficits in attention, memory, and verbal abilities may become apparent only later in development; these impairments after pediatric TBI may also manifest as an increased likelihood of needing special education programs.
The brain is rapidly developing during the first years. Events refining neural connectivity are actively taking place through processes such as myelination, dendritic changes, and synaptogenesis. As suggested by the animal literature, TBI in young children jeopardizes ongoing developmental processes. Studies by Anderson and colleagues indicate that executive functions, such as information processing and the ability to suppress impulsive behaviors, are particularly affected in those children who suffered a TBI before the age of 3 years. Deficits in executive functions were observed regardless of injury location ; thus suggesting that the alteration of neural networks caused by injury had an effect on skills that are acquired at a future developmental period. With the exception of attentional control, it was the skills that were in the process of being acquired at the time of injury that were more likely to show impairments. A recent study of young adults who had suffered from a TBI during childhood indicates that these impairments may be long lasting, particularly after a severe TBI. In these individuals quality of life and employment opportunities were reduced.
Timing of return to activity
As discussed earlier, there is substantial evidence that neural activation and plasticity are altered after developmental TBI. It is also known that physiologic neural activation can promote recovery, whereas excessive activation may exacerbate cellular damage. These neurobiological principles, then, underlie the clinically relevant determination of the optimal timing for return to activity after TBI/concussion.
Experimental
Ideally exercise should facilitate the capacity of the brain to compensate for insults, thus resulting in a better outcome. Numerous studies have shown that exercise, particularly of a voluntary nature, increases markers of neuroplasticity and promotes neurogenesis. However, this situation may not always be the case if particular considerations are not taken into account when implementing exercise in the postconcussive setting.
Animal studies have shown that the beneficial effects of exercise are found only if exercise is delayed after TBI. In these studies, rodents underwent a mild FPI and were allowed to exercise for a week, starting on the day of the injury. Not only did these rats fail to show an increase in BDNF and other target proteins but also presented cognitive deterioration when compared with their sedentary counterparts. Moreover, experimental findings suggested that premature exercise disrupts restorative processes. Proteins associated with plasticity that were increased as a result of TBI, in areas remote from the site of injury, were reduced when rats were acutely exercised. Compensatory or restorative responses may be observed in areas of the brain that have endured a lesser amount of harm.
In contrast to acute postinjury exercise, when exercise was delayed after an FPI, increases in BDNF were observed. This exercise-induced increase in BDNF was associated with enhanced hippocampal-dependent learning and memory. A cognitive benefit is typically observed as a result of voluntary exercise. In addition to BDNF, exercise increases other proteins that enhance neural function and cognitive performance. Some of these proteins have been found to increase with exercise after experimental brain injury. For example calcium-calmodulin-dependent protein kinase II (CaMKII) and cyclic adenosine monophosphate response-element-binding protein (CREB) are increased when postinjury exercise is delayed. Both of these have substances been shown to facilitate LTP, a key cellular mechanism underlying synaptic plasticity and learning. In addition, animal studies suggest that this time window is dependent on the particular characteristics of the injury; for example, the necessary delay for exercise to be effective after an FPI is severity dependent.
Although it is still unknown why the injured brain does not respond to acute postinjury exercise it is plausible that untimely exercise may divert cerebral metabolism from needed functions such as energy restoration and production of synaptic plasticity molecules, by introducing an increase in metabolic demand at a time when the brain is energetically compromised. TBI results in mitochondrial dysfunction and decreases in blood flow that compromise neuronal functioning and signaling. These disruptions in cellular function along with injury-induced changes in activation are likely to interfere with the effects of exercise.
In addition, as indicated earlier, TBI disrupts the regulation of stress hormones, and may heighten the stress response for the first postinjury weeks. Glucocorticoids, which are known to suppress levels of BDNF and other key proteins, may have increased levels after injury and contribute to the undesired effects of early postinjury exercise. In addition, a hyperresponse to stress is likely to magnify regular metabolic effects of exercise. Exercise by itself places an energetic demand and moderately increases levels of corticosteroids. Some forms of exercise elicit a stronger stress response. Different forms of exercise involve distinct motivational and fatigue characteristics. Thus certain exercise regimens with stronger stress responses may be particularly counterproductive during the early postinjury period.
Human Studies
As in animal studies, neuroprotective qualities have been associated with exercise in humans. There is a wealth of studies indicating the beneficial effects of exercise on cognitive function and mental health. In children, exercise is associated with improved academic performance and executive functioning. Exercise has also been found to decrease headaches, which are one of the main symptoms reported after mild TBI in both children and adults.
The effects of exercise have also been explored in the TBI population. A retrospective study that relied on self-reports found that postinjury exercise decreased negative mood states in adults. Affective disorders are prevalent in the population with TBI. Another study found that the practice of aquatic activities, at least 12 months after TBI, decreased the incidence of negative moods. Although most of the exercise studies assessing postconcussive effects have been in adults, it is also likely that the benefits of exercise are present in a younger population. A review of different trials with patients after TBI ranging in age from 3 to 20 years found a short-term improvement in self-esteem. Other studies have found that exercise lessens postconcussive symptoms, such as headaches, dizziness, and fatigue. Gagnon and colleagues’ study used gradual and controlled exercise rehabilitation in a pediatric population with TBI who presented symptoms 4 to 18 weeks after TBI.
Exercise studies in the population with TBI have included a wide range of postinjury periods, but few offered observations acutely after injury. Among these, a retrospective cohort study by Majerske and colleagues found that high levels of cognitive and physical activity during the early postinjury period had a negative effect on cognitive function and were associated with worsened concussive symptoms and performance on cognitive testing. The occurrence of negative mood states and cognitive impairments may be associated with neuroendocrine alterations that are found in the early post-TBI weeks, such as increased plasma cortisol levels.
These findings indicate that premature neural activation alone, without a repeated injury, can have a deleterious effect. Thus it is not only the risk of a repeated concussion that is of concern when addressing the return to play but also the potential deleterious effect of excessive, early neural activity. When a second concussion occurs the brain is more susceptible to damage as a result of ongoing conditions from the first injury. Because of these concerns, consensus guidelines for the reinstatement of physical activities after TBI recommend avoiding activities such as physical and cognitive exertion until postconcussive symptoms have resolved. Determining the optimal time for return to activity can be challenging in the sports or military setting. Having fixed waiting guidelines may be too conservative or not sufficient, because injury characteristics and recovery vary greatly between patients. However, symptom resolution may not always be self-reported accurately, nor may it reflect full cerebral or neurocognitive recovery. The timeline for effective exercise therapy as well as return to physical activities is dependent on the characteristics of the injury as well as previous injury history and thus should be tailored on an individual basis. Because of these concerns it is important to understand the physiologic and neurobehavioral correlates that underlie the injury. The literature regarding therapeutic exercise is largely based on anecdotal data and randomized studies on patients with TBI are sparse, indicating a pressing need for further rigorous investigations.
Axonal injury
Experimental
It is well known that biomechanical forces applied to neural tissue result in dysfunction and damage to axons. Changes in axonal integrity and function have been described in experimental models of mild TBI, including a recently described model of repeat concussive injury in the juvenile rat. In this juvenile model, the degree of axonal damage and glial reactivity was amplified when 2 closed head injuries were experienced 1 day apart. Behaviorally, a single mild TBI caused a decrement in working memory that was worsened by the combined injury.
Another consideration when investigating axonal damage during development is that myelination is ongoing in many brain regions throughout cerebral maturation. There is evidence that unmyelinated fibers may be more vulnerable to TBI, as shown by Reeves and colleagues. In that study, midline FPI resulted in a loss of compound action potentials through the corpus callosum, and, although recovery of the myelinated fibers occurred over time, persistent deficits in the unmyelinated peaks were seen. These data suggest that unmyelinated axons, and thus immature axons, could be more vulnerable to biomechanical injuries.
Imaging of animal TBI models provides insight into axonal injury. For example, DTI, an advanced MRI technique that measures directional diffusion as a marker of white matter fiber integrity, has been studied after experimental controlled cortical impact injury. Changes in DTI signal have been correlated with histopathologic findings.
Human Studies
In the adult brain, there is substantial pathologic evidence for axonal injury after severe TBI, but only a few such reports of axonal damage after milder, concussive injury, which were in older persons. Advances in noninvasive neuroimaging, particularly DTI, have provided new insight into white matter damage or dysfunction occurring after TBI, including in the pediatric and young adult population. Fractional anisotropy (FA) is a measure of the directionality of water diffusion, with a value of 1 being the highest, and a value of 0 indicating diffusion equally in all directions (essentially no directionality).
In children with moderate to severe TBI compared with orthopedic controls, DTI showed abnormalities in frontal white matter 3 months after injury. These abnormalities, such as decreased FA, were predictive of long-term global outcomes. Similar reductions of FA were reported in the corpus callosa (CC) of adolescents after moderate to severe TBI, which was also correlated with reduced CC NAA on MRS.
Studies of DTI after mild TBI/sports concussions have been limited to older adolescents and adults (for review of DTI methods in general, see Ref. ) An early pediatric DTI study compared control subjects with those with mild and moderate TBI (average age 15.1 ± 2.3 years, postinjury time 8.2 ± 2.2 months). This study showed lower FA values in children with moderate TBI and neurocognitive impairments in the combined mild to moderate TBI group. Specifically in the supracallosal region, both mild and moderate groups separately showed significantly reduced FA compared with controls. Wilde and colleagues measured DTI in adolescents within the first week after concussion and showed increased FA in corpus callosum, which correlated strongly with the clinical scores on the Rivermead post concussion symptom questionnaire (RPCSQ). This increased FA was postulated to indicate early axonal swelling, which favors diffusion along the direction of the callosal fibers. Using multimodal imaging combining DTI and magnetoencephalography (MEG), a group of young military and civilian adults with postconcussion symptoms and normal conventional neuroimaging were studied. This study showed areas of decreased FA in subcortical white matter underlying areas of focal slowing on MEG and supported the usefulness of this combined imaging methodology to distinguish between patients with mild TBI and controls.
It is likely that both DTI and MRS of white matter fibers will yield greater insight into postconcussion axonal pathobiology. Such noninvasive scans may be readily conducted in humans, and translational work investigating MRI and histopathology in experimental animal models will greatly aid in the elucidating mechanisms underlying abnormal white matter signals on advanced MRI sequences. Further critical work is also required in establishing clinical correlates of these white matter abnormalities, determining the longitudinal time course of axonal injury and distinguishing differences in traumatic axonal injury between the pediatric and adult brain.
Chronic traumatic encephalopathy and late risk of dementia
Chronic traumatic encephalopathy is a progressive neurodegenerative disease found in some individuals subjected to repetitive mild TBI. Neuropathologically, it can be described as a tauopathy of the brain manifesting as neurofibrillary tangles throughout most of the brain with a relative paucity of β-amyloid deposition.
Experimental
Single and recurrent mild TBI and the effect on amyloid proteins associated with dementia have been extensively studied in animal models. Most research has focused on Aβ-amyloid accumulation and its correlation with cognitive impairment. Increased levels of amyloid precursor protein (APP) have been found in animals undergoing recurrent TBI, as well as increased levels of β-secretase and γ-secretase enzymes, which are both known to convert APP into neurotoxic Aβ peptides. Likely related to these findings, Aβ1-40 and Aβ1-42 levels have also been found to be significantly increased in the hippocampal neurons of PDAPP transgenic mice overexpressing mutant human APP who are subjected to cortical impact brain injury compared with noninjured transgenic mice. The injured hippocampi showed substantial exacerbation of neuronal death, which suggests that there is a mechanistic link between brain trauma, Aβ levels, and neuronal cell death. With repetitive mild TBI in Tg APP695swe (Tg2576) transgenic mice that overexpress mutant APP, accelerated deposition of Aβ plaques and earlier onset of cognitive impairment are also seen. Research with nontransgenic mice has shown that after controlled cortical impact, accumulation of mouse Aβx-40 peptide was noted after 1 day, with increasing levels of almost 120% seen by 3 days, and normalization of levels by 7 days. Other evidence supporting the involvement of Aβ plaque formation in long-term outcomes comes from research performed by exposing pigs to inertial brain injury, which was found to cause long-term (up to 6 months after injury) accumulation of APP, β-site APP cleaving enzyme, presenilin 1, and activated caspase in the axons of injured neurons. The accumulation of these factors is believed to be caused by impaired axonal transport because of trauma, and it may lead to APP proteolysis and Aβ formation within the axonal membrane compartment. Postconcussive Aβ plaque formation may help to explain the increased risk of Alzheimer disease after mild TBI through acceleration of the postulated amyloid cascade.
Gross Pathology
Individuals with a history of multiple episodes of mild TBI may have 1 or several stigmata associated with repetitive concussion. Often seen are such findings as an anterior septum pellucidum cavum, as well as posterior fenestrations in the ventricular system. Individuals with septum pellucidum cavum may also develop recurrent obstructive hydrocephalus as a result of abnormalities in cerebrospinal fluid (CSF) flow, requiring ventricular fenestration or ventriculoperitoneal shunt placement. Other gross pathologic conditions includes atrophy of the frontotemporal cortex, medial temporal lobe, hypothalamic floor, and mammillary bodies, as well as enlargement of the third and lateral ventricles, hippocampal sclerosis, and pallor of the substantia nigra. A reduction in total brain volume may be seen, with atrophy noted in the cerebrum, diencephalon, basal ganglia, and brainstem.
Microscopic Pathology
Several different microscopic findings are seen with repetitive chronic mild TBI, including tau-positive neurofibrillary tangles, astrocytic/glial tangles, and neurites of spindle and threadlike shapes. Deposition of β-amyloid occurs in less than half of cases, according to 1 retrospective study. In addition, recent attention has been drawn to the potential involvement of transactive response (TAR) DNA binding protein-43kDa (TDP-43) in the pathophysiology of chronic traumatic encephalopathy. Diffuse cerebral white matter degeneration has long been known to occur with severe dementia after head injury. Multifocal axonal injury with damage in the corpus callosum and the fornices has been rarely described after single mild TBI in patients who died shortly after injury as a result of nonneurologic complications. Gross fornix degeneration has also been noted in similar patients.
Tau-based Protein Deposits
Historically, neurofibrillary tangles, mostly comprised of tau protein, have been regularly seen in the brains of patients with a history of multiple mild traumatic brain injuries. The exact mechanism that leads to their accumulation is not known. As mentioned earlier, shearing forces acting on neuronal axons lead to increased cell membrane permeability, ionic disequilibrium, and often trigger intracellular apoptotic enzymes, which may initiate and later aggravate formation of neurofibrillary tangles via tau truncation, phosphorylation, and misfolding. Also contributing to their production and accumulation is cytoskeletal element breakdown, notably microtubules and microfilaments.
Neurofibrillary tangles are seen predominantly in the superficial cortex (layer II and the upper portion of layer III), mostly in frontotemporal and insular distributions. They are generally found in patches, notably in the deeper portions of the sulci as well as subpial, periventricular, and perivascular areas. They are often seen with neuritic threads, seen in the subcortical nuclei/basal ganglia, hippocampus, brainstem nuclei, and cerebral cortices, with notable cortical involvement of the frontal and temporal lobes, as well as the insula. According to McKee and colleagues, both neurofibrillary and glial tangles were found in the medial temporal lobe, diencephalon, brain stem, and basal ganglia, as well as subcortical white matter of subjects with chronic traumatic encephalopathy. The perivascular distribution of neurofibrillary tangles suggests that mechanical disruption of the cerebral microvasculature may play a role in their deposition. These distributions remarkably contrast those of Alzheimer disease, which are generally seen in a more uniform cortical distribution, mostly in cortical layers III and V, without perivascular predominance.
Extension of the tau-based neurofibrillary tangles from the isolated perivascular spaces to broader, patchy distributions is not completely understood; however, some evidence suggests misfolded tau proteins are able to propagate out of 1 cell and into another, similar to prions. This phenomenon has been observed in vitro and is described as extracellular tau aggregate being taken up by cultured cells and internalized, leading to fibrillization of new tau proteins intracellularly via displacement of tubulin and colocalization with dextran.
β-Amyloid (Aβ) Deposits
Neuritic β-amyloid plaques are seen in slightly less than half of all cases of chronic traumatic encephalopathy. Their distribution differs markedly from those found in Alzheimer disease, because patients with multiple concussions show diffuse plaques with less density than the abundant plaques seen in Alzheimer disease. Although the pathogenesis of β-amyloid deposits is not entirely clear, APP has been found to accumulate in the axonal bulbs of injured neurons after diffuse axonal injury, as seen in mild TBI, most commonly in subcortical and deep white matter. APP is then likely cleaved to form β-amyloid. There is conflicting evidence regarding the exact role played by β-amyloid in pathogenesis versus neuroprotection in injured neurons.
TAR TDP-43
A recent study reported neuropathologic evidence for the theoretic epidemiologic linkage between head injury and amyotrophic lateral sclerosis. A small number of cases with chronic traumatic encephalopathy and severe motor neuron disease were found to have TDP-43 deposits in the brain as well as in the anterior horns of the spinal cord. The conclusions drawn from these results, most notably a link between repetitive mild TBI and amyotrophic lateral sclerosis, remain controversial.
CSF Markers of Repetitive Head Trauma
Short-term biochemical aberrations in the face of head trauma have been investigated. CSF markers of repetitive head trauma (neurofilament light protein, total tau, glial fibrillary acidic protein [GFAP], phosphorylated tau, and β-amyloid protein 1-40 and 1-42) have been studied in amateur boxers at 7 to 10 days and 3 months after fight and compared with healthy, nonathletic controls. Neurofilament light protein, total tau, and fibrillary acidic protein were found to be increased shortly after fights compared with 3 months later in boxers, with the increase dramatically higher among those receiving more punches or more high-impact hits to the head. Compared with controls, the boxers had significantly higher levels of neurofilament light protein and GFAP 7 to 10 days after a fight. Neurofilament light protein was the only CSF marker found to be significantly increased 3 months after a fight in boxers compared with controls.
Other CSF biomarkers have been identified and found to be altered long-term in those with repetitive head trauma. Zetterberg and colleagues compared CSF levels of S-100B, BDNF, heart-type fatty acid binding protein, GFAP, and neuron-specific enolase using biochip array in amateur boxers after 2 months of boxing abstention compared with healthy controls with no history of head trauma. These investigators found that only neuron-specific enolase was significantly increased, whereas the remainder of the CSF markers tested showed no differences between the boxers and controls. Finding no significant differences in CSF tau concentrations between resting boxers and controls supports CSF tau as a biomarker of acute concussive injury and not chronic repetitive mild brain trauma. Alterations in neurofilament light protein were not mentioned in the 2009 study.
Genetic Susceptibility to Chronic Traumatic Encephalopathy
In addition to the neuropathologic similarities between chronic traumatic encephalopathy and Alzheimer disease mentioned earlier, the genetics predisposing to both disorders also seem to have significant overlap. It is well known that apolipoprotein E is a susceptibility gene for late-onset familial and sporadic Alzheimer disease, and that possessing the ε4 genotype leads to dose-dependent hastening of the onset of Alzheimer disease. The development of chronic traumatic encephalopathy has also been linked to the ε4 genotype. Having the same apolipoprotein E ε4 genotype also predisposes the individual to incurring significantly more severe chronic traumatic encephalopathy compared with those without the ε4 genotype, given the same degree of chronic TBI. It is unknown whether possessing the apolipoprotein E ε2 genotype is protective in chronic traumatic encephalopathy, as it seems to be in Alzheimer disease. The implications of these findings support the theory that chronic traumatic encephalopathy, as well as Alzheimer disease, share a complex combination of both environmental and genetic risk factors. Future consideration should be given to the possibility of genetic counseling for professional athletes likely to encounter repeated head trauma and their likelihood of developing an encephalopathic state.
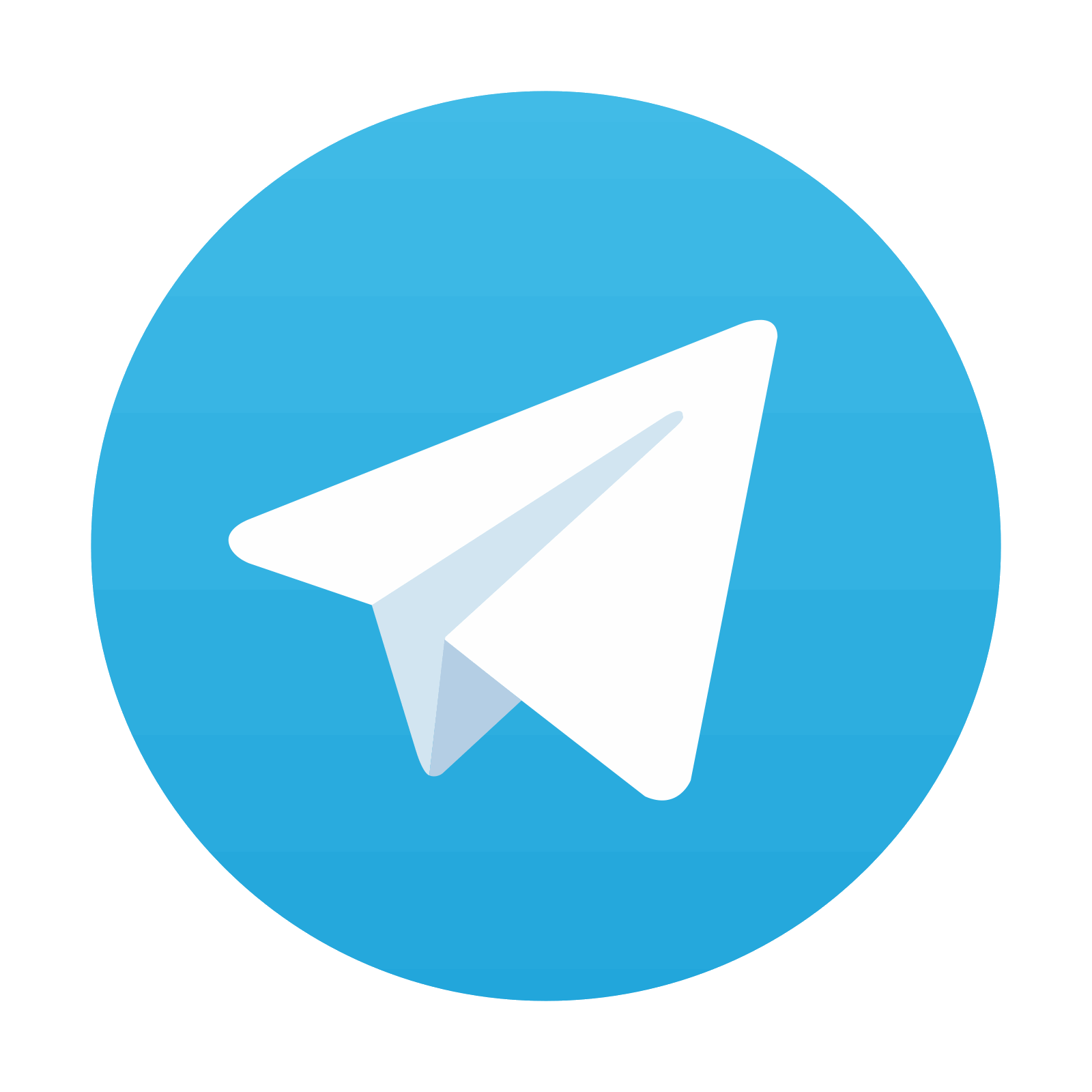
Stay updated, free articles. Join our Telegram channel
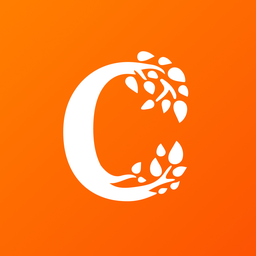
Full access? Get Clinical Tree
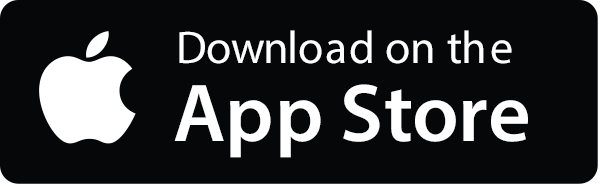
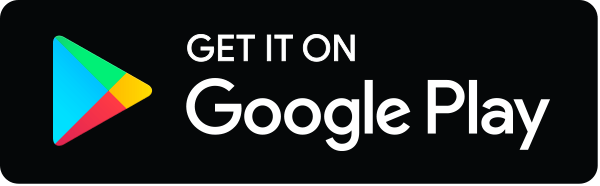