Apoptosis is a discrete form of cell death induced by multiple stimuli and mediated by a group of cysteine proteases known as caspases. This type of cell death is characterized by the degradation of nuclear DNA and is generally non-inflammatory.
Apoptosis and caspase activation can be triggered extra-cellularly by engagement of death receptors, or intrinsically by mitochondrial damage and release of cytochrome- c and Smac/DIABLO, which function to activate caspases at the apoptosome.
The Bcl-2 family includes both pro- and anti-apoptotic proteins that control cell death by regulating the mitochondrial outer membrane potential (MOMP).
Necrosis and necroptosis are both death processes that involve swelling of cells and rupture of the plasma membrane, resulting in inflammation.
Necroptosis is a specialized form of necrosis that is initiated by death ligands and regulated by signaling pathways that are also important for the regulation of apoptosis.
Nod-like receptor (NLR) molecules act as cell stress sensors to trigger inflammasome assembly and activation of caspase-1 to mediate the processing of the pro-inflammatory cytokines IL-1β and IL-18 and the cleavage of Gasdermin-D to trigger formation of a membrane pore, through which active IL-1β and IL-18 are released and pyroptosis is induced.
Autophagy is a normal cellular response to nutrient deprivation that is characterized by the degradation and recycling of cellular components.
Introduction
Cell death can proceed by one of several distinct pathways in response to signals arising from intra- and extra-cellular stresses such as DNA damage, metabolic disruption, or infection. The removal of damaged or redundant cells is critical for development, tissue turnover, and restoration of homeostasis following tissue damage or disease—but once destined to die, why does it matter how the cell engages this death?
It turns out that not all cell deaths are created equal, at least in the context of immune function. A dead or dying cell can modulate the immune response via multiple mechanisms, including the release of cytokines and chemokines. Furthermore, it is the specific type of cell death engaged that determines the nature of those signals and whether or not that cell death process is non-inflammatory or pro-inflammatory—or even, in some cases, actively suppresses inflammation. In this review, we will introduce several different types of cell death and discuss the defining features of each. The impact of these cell death pathways on immune function will be discussed in the context of disease pathologies associated with aberrant immune regulation and defects in cell death pathways.
Types of Cell Death
Apoptosis
Perhaps the best characterized of the cell death pathways is apoptosis (type I cell death), characterized by key phenotypic characteristics including perturbation and blebbing of the plasma membrane from which small membrane bound vesicles, so-called “apoptotic bodies,” are released. The plasma membrane maintains its integrity but undergoes lipid reorganization to externalize phospholipids on the cell surface. This particular event is critical with regard to how apoptotic cells are detected by the immune system. Within a cell dying by apoptosis, chromatin condenses and internucleosomal DNA cleavage proceeds, before which the dying cell then begins to shrink and, if adherent, detaches from neighboring cells and surrounding stroma and matrix.
These changes are mediated by the induced enzymatic activity of a family of cysteine proteases, the caspases that mediate the apoptotic demise of the cell and, as such, caspase activation represents one of the most universal and informative descriptive biochemical features of apoptotic cell death. There are several different caspases, each activated and regulated by different signaling pathways so that apoptotic cell death can be engaged in response to multiple types of damage or stress.
Once caspases have cleaved their target substrates, the now apoptotic cell is rapidly removed from the body via phagocytosis. Therefore, in contrast to other types of cell death, apoptosis generally, but not always, fails to evoke an inflammatory response. Even so, the term “immunologically silent,” often used to describe apoptosis, is somewhat misleading. Following their engulfment by phagocytes, apoptotic cells can remain a source of signals able to regulate components of immune function.
Necrosis—Classic or Noncanonical?
Necrosis (type III cell death) is likely familiar to most as a nonprogrammed, disordered type of death, characterized by swelling of both the cell and its intra-cellular organelles, rupture of the plasma membrane, and release of intra-cellular contents. Such death can be triggered by stresses such as altered tonicity, heat, or physical trauma, and by intra-cellular events such as Ca 2+ efflux or a rapid decrease in adenosine triphosphate (ATP) levels. “Programmed necrosis” or necroptosis is similarly characterized by rupture of the plasma membrane but is fundamentally distinct from “classic” necrosis, in that it is mediated by specific signaling pathways. Nonetheless, cellular rupture, irrespective of associated signaling events, releases numerous immune activators or danger-associated molecule patterns (DAMPs) to generate a potent inflammatory response.
Features typically associated with apoptosis can be detected in cells undergoing necroptotic death, including the externalization of inner membrane phosphatidyl-serine, partial chromatin condensation, and phagocytic uptake. The requirement for caspase activity is generally used to discriminate apoptosis from necrosis (but see pyroptosis, discussed in the following section). However, caspase activity, critical for apoptosis, is not only dispensable for necroptotic cell death but in some cases actually requires the active inhibition of one specific caspase for programmed necrosis to proceed (discussed later).
Necroptosis can be induced by death receptors of the TNF superfamily, members of the immune sensing Toll-like receptors (TLRs), and as part of the interferon response. Accordingly, a close, and in some cases overlapping, functional relationship, between signaling components of the immune system and those of necroptosis has emerged. Several components of the upstream death receptor signaling machinery are common to both apoptosis and necroptosis but, downstream, specialized death pathway-specific adapters ensure the appropriate engagement of each form of cell death. For example, in the case of necroptosis, the critical effector molecules are the receptor interacting protein kinase 3 (RIPK3) and its substrate, mixed lineage kinase domain-like (MLKL).
Pyroptosis
Pyroptosis, also a form of regulated necrosis, is mediated by the catalytic activity of the “inflammatory caspases,” which include caspase-1 and, under some circumstances, caspase-4 and caspase-5 (caspase-11 in rodents). , Pyroptosis is mediated by one caspase substrate, which causes cellular lysis that is preceded by a marked increase in cell size, attributed to a caspase-dependent formation of membrane pores, which is thought to provoke a consequent disruption of ionic gradients across the cell membrane and an increase in osmotic pressure that causes water influx, cell swelling, and membrane rupture. There is, however, evidence that additional steps are required for full cell lysis. The resulting release of intra-cellular contents provides a localized pool of potential pro-inflammatory molecules that include both direct activators of immune cells as well as so-called “danger signals” that trigger the production of inflammatory molecules from other cells. Because caspase-1 (but not caspase-4, -5, or -11) can also cleave and activate executioner caspases (e.g., caspase-3 and caspase-7, see later), caspase-1 activation can induce apoptosis when the substrate responsible for pyroptosis is absent.
Autophagy
Autophagy is a catabolic process in which cellular components are enclosed within a double membrane bound vesicle or autophagosome, before being targeted to lysosomes for degradation. Autophagy plays a role in cell survival by providing energy and metabolites during times of starvation and for the removal of damaged organelles and long-lived proteins. It may also engage cell death (type II cell death), particularly under conditions in which autophagy is enforced. However, the lack of stringent experimental discrimination between autophagy-induced cell death and autophagy arising as a consequence of cell death has proven a rich source of controversy. Consequently, the term “autophagic cell death” must only be applied from a functional perspective and only to scenarios in which cell death can be inhibited by the suppression of key regulators of autophagy. This excludes most examples in mammalian systems, and our understanding of autophagic cell death is limited, with very little insight into the physiologic conditions under which it is engaged. While autophagy regulates cell death pathways as well as immune responses, we do not consider it further herein.
Molecular Mechanisms of Caspase Activation
Caspases and Apoptosis
Apoptotic cell death is executed by one or more caspases, a family of cysteine proteases with selectivity for aspartate (Asp/D) residues that cleave their target substrates after tetrapeptide sequences containing aspartic acid at the P1 position, for example, DEVD. All caspases are synthesized as inactive monomeric zymogens with an N-terminal prodomain and a C-terminal protease domain, consisting of a large and a small subunit and the catalytic cysteine residue, require dimerization, in addition to other events for catalytic competence.
Caspases are categorized according to their general function. The executioner caspases, caspases-3, -6, and -7, are responsible for the cleavage of substrates to effect cellular destruction and clearance of the dead cell. In contrast, the initiator caspases, including caspases-8 and -9 (and caspase-10 in humans) provide the molecular “ignition” to trigger the proteolytic cascade and facilitate executioner function. The inflammatory caspase, caspase-1, can also trigger apical events; although its role is largely in immune regulation, it can participate in apoptosis under some circumstances (noted previously).
Caspase Substrates of Note
The initiator caspases are responsible for two critical cleavage events: (1) autocleavage to induce stabilization of the active dimer (caspases-8 and -10) or destabilization (caspases-9 and -1); and (2) the cleavage and activation of the executioner caspases-3 and -7 that can, in turn, cleave and activate another executioner caspase, caspase-6. It is therefore important to note that while proper cleavage of an executioner caspase is evidence of its activation, cleavage of an initiator caspase is not necessarily evidence of its activation.
Once active, executioner caspases cleave numerous target substrates, including inhibitor of caspase-activated DNase (ICAD), which inhibits active CAD, the caspase-activated DNase responsible for DNA fragmentation, , poly-ADP-ribosyltransferase (PARP), a DNA repair enzyme, components of the cytoskeleton and matrix, and targets responsible for phosphatidylserine externalization, critical for phagocytic uptake and removal of dead cells.
The inflammatory caspase, caspase-1, cleaves two pro-inflammatory cytokines, IL-1β and IL-18, to generate bioactive cytokines that are released upon pyroptosis. Unlike caspase-1, the other inflammatory caspases-4, -5, and -11 do not cleave the proforms of these cytokines. Additional caspase-1 substrates include components of the actin cytoskeleton, the cellular inhibitor of apoptosis proteins (cIAPs), and metabolic enzymes, although the relevance of these cleavage events to caspase-1-dependent cell death remains poorly characterized. Gasdermin-D (GsdmD) is cleaved by all of these inflammatory caspases (but not executioner caspases), and upon cleavage forms pores in the plasma membrane responsible for pyroptosis.
Another caspase substrate effectively blurs the distinction between apoptosis and necrosis. Like GsdmD, another member of this family, GsdmE, is capable of forming destructive plasma membrane pores upon its cleavage. However, GsdmE is cleaved by caspase-3 and caspase-7 (and not inflammatory caspases) and, therefore, cells that express GsdmE, upon induction of apoptosis, have features of both forms of cell death (this has been called “secondary necrosis”). ,
Caspase Regulation: Activation and Inhibition
Initiator and executioner caspases require different mechanisms of activation. Initiator or apical caspases have long prodomains and exist as inactive monomers that must be dimerized to generate and stabilize the catalytic site. This is mediated by the formation of oligomeric signaling complexes, comprising the apical caspase, specialized adapter proteins, and additional regulatory proteins. In contrast, the executioner caspases exist as preformed inactive dimers with minimal prodomains that are rendered active by enzymatic cleavage of a DEVD site within the linker region between the large and small subunits. In this way, dimerization of an apical caspase, enforced by recruitment to its specific activation complex, generates an active enzyme that, in turn, cleaves and activates downstream executioner caspases to mediate proteolysis of specific target substrates and cellular destruction.
Caspase Inhibitors (IAPs)
Inhibitor of apoptosis proteins (IAPs), characterized by one or more copies of a motif called the baculovirus IAP repeat (BIR), can bind to and suppress active caspases. First identified in viruses, IAPs ensure viral replication by inhibiting caspase activity in the host cell to sustain cell survival. Subsequently, BIR-containing proteins were identified in vertebrates that included XIAP, c-IAP1, c-IAP2, NAIP, , and survivin.
It is only XIAP that directly blocks apoptosis by interacting with and inhibiting active caspases. XIAP is characterized by three BIR domains, with BIR3 and adjacent RING domain mediating the inhibition of caspase-9 and BIR2 and adjoining linker region suppressing the executioner caspases-3 and -7. XIAP, as well as c-IAP1 and c-IAP2, can function as E3-ligases to promote ubiquitination and proteasomal degradation of their target protein substrates. In this way, XIAP can also halt caspase activity by inducing protein degradation. Although XIAP-deficient mice have no obvious developmental defects, there are certain circumstances in which XIAP may be a critical determinant of cellular sensitivity to certain apoptotic pathways (discussed in more detail later).
Playing CARDS (and DEDs)—Activation Platforms of the Initiator Caspases
The recruitment of apical caspases to large protein complexes is determined by the specialized adapter molecules within these complexes and the reciprocal binding domain within the caspase itself. The prodomain of the initiator caspases contains one of two specialized binding domains, the CARD (caspase recruitment domain), present in caspases-1, -2, -4, -5 -9, -11, and -12, and the DED domain, found in caspases-8 and -10. The DED and CARD domains display no sequence homology, although structurally they are similar in that they form so-called “death folds.” The death domain (DD) and pyrin (PyD) domains, although not a structural feature of caspases themselves, are found in other proteins that participate in caspase activation.
The Inflammasome—Activation Platform for Procaspase-1
Caspase-1 is the prototypical human inflammatory caspase and exists as an inactive monomer that is activated by its CARD-dependent recruitment to a multiprotein complex, the “inflammasome.” Caspase-1 is essential for the processing of the inactive proforms of IL-1β and IL-18 to generate active cytokines, the release of which occurs via a noncanonical secretory pathway that also requires caspase-1 function. Initiation of caspase-1-dependent IL-1β/IL-18 release is most often associated with components of pathogenic organisms, collectively termed pathogen-associated molecular patterns (PAMPs), which function by engaging members of the Nod-like receptor (NLR) family in the cytosol (discussed in the following section).
The DISC—Activation Platform for Procaspase-8
Activation of caspase-8 and caspase-10 (found in humans and not rodents) is triggered via death receptors, a subset of the TNF receptor family when ligated by their cognate death ligand, also TNF-like molecules. Depending upon which accessory protein is recruited to the signaling complex, procaspase-8 cleavage and/or activation can participate in one of several biologic pathways.
Constitutively trimerized death receptor is activated by trimers of death ligand to form homotypic DD-DD interactions with one of several adapter molecules including FADD, to reveal the DED domain and recruitment of procaspase-8 or procaspase-10 via one of their two DED domains. Enforced dimerization and activation of these procaspases promotes the cleavage and activation of the effector caspases-3 and-7. That is, unless procaspase-8 forms heterodimers with a catalytically inert homolog, c-FLIP L (herein, FLIP L ), a negative regulator of procaspase-8 activity. FLIP-procaspase-8 heterodimers, although lacking the ability to promote apoptosis, retain sufficient catalytic activity to inhibit an alternative form of cell death, necroptosis, which is discussed in more detail later.
The Apoptosome—Activation Platform for Procaspase-9
Procaspase-9 activation is mediated via its recruitment to a large protein complex called the apoptosome, comprising procaspase-9, APAF-1 (apoptotic protease activating factor-1), and additional regulatory proteins. , APAF-1 is a specialized adapter protein containing an N-terminal CARD domain and a C-terminal protein-binding domain consisting of multiple WD repeats. APAF-1 is a cytosolic molecule, triggered to undergo self-oligomerization by the binding of cytochrome- c following its release from the mitochondrial intermembrane space. Association with cytochrome- c , together with the binding of deoxynucleotides (dATP), unmasks the C-terminal CARD domain of APAF-1, allowing it to interact with the CARD domain of procaspase-9. Once activated, caspase-9 then cleaves to activate the executioner caspases-3 and -7 .


Mitochondria and Apoptosis—INTRINSIC Apoptosis
Caspase-dependent apoptosis is broadly defined as two pathways that, for the most part, proceed independently of one another—the mitochondria-dependent “intrinsic” pathway and the “extrinsic” pathway that is mediated by death receptor/death ligand interaction. The former, dependent upon the initiator caspase-9, is characterized by mitochondrial outer membrane permeabilization (MOMP) to release cytochrome- c , an intermembrane component of the electron transport chain, into the cytosolic space. Once released, cytochrome- c binds to APAF-1 to facilitate assembly of the apoptosome and activation of procaspase-9 (see previous). Several additional proteins are also released that include SMAC (second mitochondrial-derived activator of caspase), also known as DIABLO, OMI/Htr2A, and AIF. SMAC and OMI participate indirectly in caspase activation through binding and antagonism of XIAP (discussed in more detail later).
Regulation of Mitochondrial Outer Membrane Permeabilization (MOMP)
MOMP is one of the primary features of the intrinsic apoptotic pathway and is positively and negatively regulated by the Bcl-2 (B cell lymphoma-2) family of proteins ( Fig. 19.1 ) (reviewed in refs. 37, 38). Bcl-2 proteins are grouped into those that promote and those that suppress cell death by directly or indirectly regulating MOMP according to specific structural features.
The proapoptotic multidomain effector proteins, BAX and BAK, directly permeabilize the outer mitochondrial membrane while the anti-apoptotic family members including Bcl-2 itself, Bcl-X L , A1, Bcl-B, Bcl-W, and Mcl-1 function primarily to prevent MOMP. The proapoptotic BH3 only proteins, including BID, BIM, PUMA, NOXA, HRK, BIK, BMF, and BAD, also promote MOMP, but do so indirectly, either by activating the effectors, BAX and BAK, or inhibiting the anti-apoptotic Bcl-2 proteins.
Monomeric BAX is found in the cytosol, while BAK resides on the outer mitochondrial membrane, anchored by its carboxy-terminal region. Conversion to their active state requires transient interaction with one or more of the BH3-only proteins to induce a change in their conformation, multimerization into large homo-oligomers and, in the case of BAX, translocation to the outer mitochondrial membrane. The subsequent release of intermembrane space proteins requires membrane permeabilization, but whether BAX and BAK oligomers achieve this directly by membrane insertion and forming pores themselves, or indirectly, by triggering the formation of lipid pores, has yet to be resolved. Nonetheless, following permeabilization, cytochrome- c , SMAC (DIABLO), and OMI (Htr2A) are released from the mitochondrial intermembrane space into the cytosol to activate caspases and mediate apoptotic cell death.
A third Bcl-2 family member, BOK, can similarly induce cell death via MOMP. BOK protein is inherently unstable and under constitutive conditions is degraded via the endoplasmic reticulum (ER)–associated degradation pathway (ERAD). Inhibition of the proteasome or various forms of ER stress leads to the stabilization and accumulation of BOK protein to promote MOMP and trigger apoptotic cell death. However, the precise role of BOK remains somewhat of a mystery—neither the ablation of BOK expression nor its co-deletion with BAX or BAK revealed any overt developmental phenotype. Interestingly, however, BOK, unlike either BAX or BAK, is frequently deleted in cancers.
Insertion of BAX or BAK into the outer mitochondrial membrane exposes the BH3 domain, allowing self-oligomerization to induce MOMP or, alternatively, interaction with an anti-apoptotic Bcl-2 protein to inhibit it. In contrast, the sequestration and neutralization of the anti-apoptotic Bcl-2 proteins by the BH3-only proteins increases the likelihood of BAX and BAK activation to induce MOMP. Specific members of the BH3 protein family, BID, BIM, and PUMA, so-called “direct activators,” are able to trigger the conversion of BAX and BAK from their constitutively inert state into active oligomers capable of inducing MOMP.
The engagement of cell death in the context of caspase inhibition can promote a type of cell death that is also characterized by MOMP and Bcl-2 protein function—so-called caspase-independent cell death (CICD). This type of cell death is perhaps most commonly observed during development and particularly under conditions in which certain pathways of caspase activation are inactivated, such as in the APAF-1 deficient model. In this case, cells die due to a bioenergetic catastrophe, as mitochondrial function is lost upon MOMP.
Post-translational Regulation of Bcl-2 Protein Function
Bcl-2 proteins are regulated at the transcriptional level, and can also be regulated by several post-translational modifications including phosphorylation, ubiquitin-mediated degradation, protease-mediated cleavage, myristiolyation, and neddylation. Although the precise functional consequences of these modifications are not clear in many cases, there are a few exceptions.
Kinase-mediated phosphorylation of the BH3-only proteins BIM and BAD is critical for the integration of upstream signaling events associated with growth factor withdrawal and activation of downstream apoptotic effectors. Phosphorylation of BIM at serine-69 by the mitogen-activated protein kinase (MAPK) extra-cellular-regulating kinase (ERK) induces the proteasomal degradation of BIM. In contrast, the phosphorylation of BIM by the MAPK, c-Jun N-terminal kinase (JNK), stabilizes the protein to facilitate BAX-dependent cell death. AKT-dependent phosphorylation of BAD at residues Ser-112 and Ser-136 promotes the association of BAD with 14-3-3, 46 while the dephosphorylation of BAD in response to nutrient deprivation neutralizes its proapoptotic activity by triggering its interaction with Bcl-X L or Bcl-2.
Multiple phosphorylation and ubiquitylation events also contribute to the regulation of MCL-1 stability. Disruption of AKT activity under conditions of nutrient withdrawal relieves the inhibition of the kinase, GSK3, which phosphorylates MCL-1 to promote its degradation. Interaction between MCL-1 and MULE, an E3 ligase, when disrupted by a proapoptotic BH3-only protein such as NOXA, allows the ubiquitlylation and degradation of MCL-1. Other proteins that control the stability of MCL-1 include FBW-7, a tumor suppressor frequently deleted in cancers, and the deubiquitinase USP9X, expressed in many tumor types. The turnover of the anti-apoptotic proteins Bcl-2 and Bcl-XL are similarly subject to regulation by post-translational modification. , For example, ERK1/2-mediated modification of a serine residue (Ser-70) within the flexible loop between the BH3 and BH4 domains or on serine-87 of Bcl-2 both exacerbate its anti-apoptotic activity, at least in part, by maintaining protein stability. Dephosphorylation by PPA2 opposes this activity and neutralizes the anti-apoptotic activity of Bcl-2. In contrast, the anti-apoptotic activity of Bcl-x L is disrupted by the deamidation of two asparagine residues in the unstructured loop between BH4 and BH1.
Specific cleavage events can also contribute to the function of certain Bcl-2 proteins. The BH3-only protein BID is unique among its BH3 relatives in that in its native state, its BH3 domain is buried within the tertiary structure of the molecule, rendering it inactive. However, cleavage of a flexible linker within BID by one of several proteases, including caspases-8 and -2, granzyme B, lysosomal proteases (cathepsins), and the calcium-activated protease, calpain, generates an active cleavage product, tBID, rendering it able to associate with anti-apoptotic proteins or function as a direct activator of BAX and BAK. In this way, upstream cytosolic events leading to the activation of these proteases can engage mitochondria-dependent apoptosis by the direct cleavage and activation of the BH3 protein, BID. Calpain-mediated cleavage of BAX to enhance its proapoptotic activity has also been described, although the physiologic significance of this event has yet to be fully delineated.
Multiple transcription factors have been implicated in the regulation of both pro- and anti-apoptotic Bcl-2 gene expression, including p53, nuclear factor κB (NF-κB), and FOXO3a. Several micro RNAs (miRNAs), including MiR-17-92, Mir-15, and Mir-16, specifically reduce the levels of BIM and Bcl-2 RNAs. Several of the Bcl-2 proteins exist as multiple splice variants, including Bcl-XL, BAX, and PUMA, although their specific roles are poorly defined. However BIM, found as multiple isoforms, including BIM-S, BIM-L, and BIM-EL, appear to harbor differing proapoptotic potencies as a consequence of the presence or absence of a dynein light chain-binding region. Anoikis, apoptotic cell death arising from perturbation of the cytoskeleton and detachment of cells from the basement membrane, is mediated by BIM and another BH3 protein, BMF. BIM is anchored to the microtubule complex, while BMF is sequestered by the actin cytoskeleton, each by their respective dynein light chain binding regions. Disruption of the cytoskeleton induces the release of BIM and BMF to promote MOMP and engage apoptosis.
Alternative/Additional Roles of Bcl-2 Proteins
Our discussion of Bcl-2 function has focused on their role in cell death in the context of MOMP regulation. Although a critically important role, it is becoming apparent that the collective activity of Bcl-2 proteins is not restricted to the regulation of cell death, but that it likely has significant impact in several additional signaling pathways including the regulation of mitochondrial dynamics, the response to DNA damage, , metabolic bioenergetics, Ca 2+ homeostasis, ER function, and autophagy.
Death Receptor–Associated Signaling Events—EXTRINSIC Apoptosis
The death ligands and their receptors exist as preformed trimers and represent subsets of the TNF and TNF receptor (TNFR) superfamilies, respectively. The receptors include TNF-R1, CD95 (also known as Fas or Apo-1), DR3 (death receptor 3), the TRAIL (TNF-related apoptosis ligand) receptors, TRAIL receptor-1 (or DR4), TRAIL receptor-2 (also known as DR5 in humans), and death receptor 6 (DR6). Their corresponding activating ligands are TNF itself, CD95-ligand (or FasL), and TRAIL, all of which engage apoptosis. Neither DR3 nor DR6 promote apoptosis. Interaction between a death receptor and its appropriate ligand induces a conformational change to expose the DD, a specialized protein-association domain in the receptor, making it available for interaction with one of several DD-containing adapter proteins.
Interaction between FasL (CD95 ligand) and its receptor Fas (CD95) triggers the recruitment of the DD-containing adapter protein FADD. This is accompanied by a conformational change in FADD to reveal a second specialized binding domain, the DED. Assembly of the mature signaling complex or CD95 death inducing complex (DISC) is completed by the recruitment of procaspase-8 via interaction between one of two DEDs in its prodomain and the DED of FADD. The consequent dimerization of procaspase-8 permits self-cleavage and stabilization of the active caspase, leading in turn to the proteolysis and activation of the effector caspases-3 and -7. DISC formation is negatively regulated by the dimerization of caspase-8 with its catalytically inactive homolog, FLIP. Although this interaction prevents the dimerization and cleavage of caspase-8, it does not completely inhibit its catalytic activity. This results in the cleavage of FLIP but, importantly, not the induction of apoptosis. Recently, studies have further characterized the complexity of binding among FADD, FLIP, and caspase-8, demonstrating it to be an important determinant of the type of cell death that proceeds, apoptosis or necroptosis (discussed in detail in the following section).
The signaling events elicited by activation of the TNFR1 are complex, subject to a variety of regulatory mechanisms, and leading to cellular survival, apoptosis, or programmed necrosis, depending upon the specific identity of the proteins recruited to the TNFR1 receptor-signaling complex, termed Complex I, IIa, and IIb (details are described in Fig. 19.2 ). TNFR1 activation can be mediated by one of two ligands, TNF itself or lymphotoxin, both of which trigger conformational changes within the intra-cellular domain of the receptor to expose the DD as well as an additional binding site for an E3-ligase, TNF-receptor associated factor-2 (TRAF2). Subsequent recruitment of TRADD to TNFR1 via their respective DD domains serves to both stabilize the TRAF2-TNFR1 interaction and recruit RIPK1. RIPK1 is a functionally versatile molecule, able to contribute to the activity of several TNRR1 signaling complexes, some of which require its kinase activity and others that do not. In Complex I, RIPK1 acts to recruit additional signaling components to the TNFR1 complex in a kinase-independent manner once modified by TRAF2-mediated lysine 63-linked polyubiquitylation. One of these is NEMO (NF-κB essential modulator), a component of the IKK (IκB kinase) complex that stimulates the phosphorylation, ubiquitylation, and degradation of IκBα to reveal the transcriptional activity of NF-κB. It is important to note that, under these conditions, cell death does not proceed, but instead NF-κB induces the transcription of genes that contribute to cellular survival, including cIAP1, cIAP2, and FLIP L , and others that participate in inflammatory responses.
Under some conditions, the TRADD-TRAF-2-RIPK1 signaling complex is released from TNFR1, where the DD domain of TRADD, available after detachment from the TNFR1, now recruits FADD. This scenario is compatible with the formation of one of two signaling complexes, termed Complex IIa and IIb. Recruitment of FLIP L to form heterodimers with caspase-8 prevents the assembly of catalytically active caspase-8 homodimers to inhibit apoptosis but in a manner that retains the ability of caspase-8 to cleave and inactivate RIPK1 and RIPK3. However, in the absence of FLIP L , the catalytic activity of caspase-8 is unrestrained and therefore able to promote apoptosis. In contrast, inhibition of caspase-8, or its requisite adapter, FADD, promotes assembly of Complex IIb, also termed the necrosome, by allowing the recruitment and stabilization of RIPK1 and RIPK3 to instead trigger necroptosis. In this way, FLIP L provides a critical point at which TNFR1 signaling events are integrated to determine the mode of cell death engaged.
Molecular Interaction With the Mitochondrial Pathway—Caspase-8 and BID
For the most part, the apical signaling events of the intrinsic and extrinsic apoptotic pathways proceed independently of one another, converging only at the terminal executioner phase of caspase-3 activation. However, MOMP, typically associated with the intrinsic death pathway, can also participate in death receptor-mediated apoptosis by virtue of caspase-8-mediated cleavage and activation of the BH3 protein, BID. XIAP appears to be the critical determinant of the relative involvement of BID-mediated MOMP in the extrinsic apoptosis pathway.
XIAP binds to and blocks the proteolytic activities of caspase-9, -3, and -7 but not caspase-8, unless displaced by SMAC and OMI, both of which are released from the mitochondria upon MOMP. Thus, when XIAP is expressed, the activation of BID and promotion of MOMP are required for death receptor-induced apoptosis, via the derepression of XIAP by SMAC and OMI.
Caspases and Pyroptosis
Assembly and activation of the inflammasome complex to initiate the production of the pro-inflammatory cytokines, IL-1β and IL-18, represents a critical component of the host innate immune system to protect against infection and sterile insults. The initiating event for inflammasome assembly is the detection of a “stressor” by one of several sensor molecules or nucleotide-binding domains, leucine-rich repeat-containing (NBD-LRR) or NLRs. Activators of NLRs, collectively termed DAMPS, can be of endogenous, exogenous, self, or foreign origin although most are commonly associated with components of infectious agents or PAMPS.
NLRs are among the so-called pattern-recognition receptors (PPRs) that encompass TLRs, C-type lectin receptors (CLRs), the absent in melanoma 2 (AIM2)–like receptor (ALR) families, and retinoic acid inducible gene (RIG)–I-like receptors (RLRs). NLR members include NLRP1 (NOD-, LRR-, and pyrin-containing domain 1), NLRP3, NLRP6, NLRP7, NLRP12, and NLRC4 (also named IPAF), and are characterized by three distinct domains. The N terminal region that confers the functional classification of the NLR contains a pyrin, caspase recruitment domain (CARD) or baculovirus inhibitory domain, the central domain, NBD or NACHT domain (nucleotide-binding domain) that mediates dNTP hydrolysis and multimerization, and the C-terminal leucine rich repeat (LRR) domain. It is the LRR domain, ascribed autoinhibitory function in several inflammasome complexes, that promotes structural stability and a variety of protein-protein interactions.
The inflammasome, although structurally analogous to the caspase-8 activating DISC and the caspase-9 activating apoptosome in general terms, differs from these caspase-activation complexes in one significant way. The inflammasome complex can vary in its molecular composition by virtue of different adapter molecules that are themselves, characterized by one of several different protein binding domains ( Fig. 19.3 ). Despite the compositional variations of inflammasome complexes, their primary role is to induce caspase activity via direct or indirect interaction with the CARD domain of caspase-1. One of the most common adapter molecules required for inflammasome assembly is ASC (apoptotic speck-forming CARD), which mediates IL-1 and IL-18 processing via homomultimeric interactions between its CARD domain and that of caspase-1. ASC also contains a pyrin domain (PyD), a death domain (DD) that mediates interaction between the PyD domain of several members of the NLR family including NLRP1, NLRP3, NLRP 6, NLRP7, NLRP12, and NLRC4 to promote inflammasome assembly. Activating ligands are derived from multiple sources and include endogenous ligands such as glucose and amyloid-β, environmental stressors including asbestos and ultraviolet (UV) irradiation and those derived from infectious agents of bacterial, viral, fungal, and protozoan origin.



The NLRP3 Inflammasome
Assembly of an active NLRP3-containing inflammasome requires, in most cases, two independent signaling events—the first primes the cells and arises from NF-κB activation mediated by TLR or TNF-R1 to induce expression of NLRP3 and other inflammasome components, while the second event is mediated by activation of NLRP3 via one of several DAMPS or PAMPs that include asbestos, amyloid-β, uric acid, muramyl-dipeptide, and calcium pyrophosphate dihydrate (CPPD) as well as bacterial RNAs and viral components derived from multiple pathogens ( Fig. 19.3A ). In response to these triggers, NLRP3, via its PYD domain, associates with the PYD-containing adapter ASC. The CARD domain of ASC then interacts with that of caspase-1 to promote inflammasome formation, the processing of IL-1β and IL-18, and activation of Gasdermin-D to induce pyroptosis.
The promiscuous nature of NLRP3 activation is likely to represent a common activation mechanism rather than multiple factors directly interacting with NLRP3 to promote inflammasome assembly. However, despite this assertion, a unifying mechanism has yet to emerge, although many potential triggers have been proposed (see Fig. 19.2A ). These include several mitochondrially localized events such as reactive oxygen species (ROS) production, oxidation of mitochondrial DNA, translocation of cardiolipin, and direct association between K63 ubiquitinated NLRP3 and mitochondrial anti-viral signaling (MAVS) proteins or mitofusin 2. Molecules that physically interact with NLRP3 to promote inflammasome assembly include double-stranded RNA-dependent protein kinase (PKR) and guanylate binding protein (GBP). Several additional regulatory factors include K + efflux, , Ca 2+ influx, , ATP-triggered puraminergic signaling events, and interaction with chaperone proteins including HSP90 and SGT1 via the LRR domain of NLRP3 to maintain a stable but inactive complex.
Multiple inherited and de novo mutations in the NLRP3 gene have been identified, many clustered within the NBD domain, rendering a genetic predisposition to increased formation of the NALP3-containing inflammasome. Increased IL-1β production contributes to the pathophysiology of a family of cryopryinopathies or cryopyrin-associated periodic fever syndromes (CAPS) including familiar cold-induced autoinflmammatory syndrome (FCAS), Muckle-Wells syndrome (MWS), and neonatal onset multisystem inflammatory disorder or chronic infantile neurologic cutaneous and articular syndrome (NOMID/CINCA). Genetic characterization of a contributory role for NLRP3 in CAPS was identified using murine knock-in models harboring specific NLRP3 mutations associated with MWS and FCAS. These NLRP3 mutations, when introduced into cells of the hematopoietic compartment, manifested in several clinical features of the human syndromes, including neutrophil infiltration into the skin, periodic bouts of fever, and elevated Th17-associated cytokines. These symptoms were largely alleviated by anti-IL-1 treatment, suggesting that the underlying pathophysiology can be attributed to increased IL-1β secretion as a consequence of the enhanced formation of NLRP3-containing inflammasomes.
Several other pathophysiologies associated with exuberant production of pro-inflammatory cytokines, including IL-1β, have also implicated NLRP3 function. NLRP3-dependent inflammasome formation can be triggered by the mislocalization of endogenous molecules including those typically associated with gout, an inflammatory disease arising from deposition of monosodium urate (MSU) crystals in the joints, cholesterol deposits in atherosclerotic plaques, and insoluble aggregates of β-amyloid, a feature of Alzheimer’s disease. Tissue damage arising from ischemic-reperfusion injury or that associated with excessive inflammation in the lung during pulmonary fibrosis or asthma is characterized by the release of multiple DAMPS including extra-cellular ATP, uric acid, the purinergic receptor P2X7, and hyaloronan, a component of the extra-cellular matrix—all of which can directly induce the formation of an NLRP3-dependent inflammasome. Furthermore, lung inflammation arising from environmental factors such as asbestos and silica may well be attributable to the reported NLRP3-dependent production of IL-1β and IL-18 triggered by these agents. Although a direct role for NLRP3 in all of these aberrant inflammatory scenarios remains to be fully tested, a contributory role in the associated pathophysiologies seems likely.
The NLRP3-containing inflammasome is an essential component of the host immune response to numerous bacterial, fungal, and viral pathogens. Examples include Staphylococcus aureus, Listeria monocytogenes, Escherichia coli, Klebsiella pneumonia, Shigella flexneri, Candida albicans, and Saccharomyces cerevisiae . Several of these organisms express pore-forming toxins that induce membrane rupture and NLRP3-dependent inflammasome activation and/or pyroptosis. These include Toxins A and B from Clostridium difficile , pneumolysin from Streptococcus pneumonia , nigericin from various strains of Streptomyces , α-, β-, and γ-hemolysins, the virulence factors from Staphylococcus aureus , and listeriolysin from Listeria monocytogenes.
Host-mediated immunity against some DNA viruses including influenza, , herpes, , and Sendai may also involve activation of the NLRP3 inflammasome. However, identification of AIM2 as the primary sensor of nucleic acids to trigger ASC-dependent inflammasome activation may limit the general role of NLRP3 under some conditions. Nevertheless, clearly NLRP3 is a critical component of effective host defense against multiple bacterial pathogens and DAMPS of self-derived origin.
The NLRC4 Inflammasome
NLRC4 itself contains a CARD domain, capable of direct interaction with the CARD of caspase-1, thereby negating the requirement for an adapter such as ASC to promote caspase-1 activation and release of IL-1β and IL-18 ( Fig. 19.3B ). Cytosolic assembly of the NLRC4 inflammasome is triggered by components of the Type III or Type IV secretion systems or by flagellate bacteria including Shigella flexneri, Pseudomonas aeruginosa, and Salmonella typhirium. NLRC4 is a unique member of the NLR family in that the mechanism mediating its response to pathogens is known. Members of the NLR-apoptosis inhibitory protein (NAIP) family, 6 paralogs in mice, function as the upstream sensors to then directly engage NLRC4 inflammasome assembly and IL-1β maturation.
Two independent NLRC4 gain-of function point mutations were recently described, both of which rendered the NLRC4 inflammasome constitutively active and were associated with periodic bouts of fever. Functional analyses revealed aberrant IL-1β/IL-18 production and an increased susceptibility to pyroptosis within macrophage populations isolated from patients.
The NLRP1b Inflammasome ( Fig. 19.3C )
NLRP1, the first NLR to be identified as an inflammasome mediator, is encoded by a single gene in humans but by three orthologs in the mouse, denoted Nlrp1a, Nlrp1b, and Nlrp1c. Unlike human NLRP1, which contains a pyrin domain, the murine paralogs do not and are composed of a nucleotide binding domain, an LRR region, a FIIND (“function to find” domain), and a C terminal CARD. Muramyl dipeptide (MDP), a minimal bioactive peptidoglycan peptide common to both positive and negative bacteria, was initially identified as a ligand for Nlrp1b-mediated inflammasome formation. However, whether Nlrp1b-mediated inflammasome formation and caspase-1 processing requires pyrin domain-dependent association with ASC or instead occurs via a direct interaction between NLRP1 and caspase-1 via their respective CARD domains remains unclear.
Lethal toxin (LT), the major virulence factor of Bacillus anthracis , is a potent ligand for Nlrp1b-dependent inflammasome formation and triggers IL-1β and IL-18 processing and extensive pyroptosis of macrophages. LT promotes the cleavage of Nlrpb1, , an event that is reportedly both necessary and sufficient for inflammasome assembly.
Interestingly, an activating mutation of NLRP1 and consequent predisposition to inflammasome formation, although associated with lethal systemic inflammation via IL-1β, also led to caspase-1 activity and extensive pyroptosis in the hematopoietic compartment independently of IL-1β production. Nlrp1a-mediated inflammasome formation and pyroptosis may therefore have additional roles independently of that of IL-1β-mediated inflammation.
The NLRP6 Inflammasome
NLRP6 is expressed predominantly in gut epithelial cells and can mediate ASC-dependent assembly of the inflammasome, caspase-1 processing, and enhanced secretion of IL-1β and IL-18. Mice lacking Nlrp6, ASC, or caspase-1 exhibited a marked exacerbation in colitis and an enhanced susceptibility to damage-associated gut tumorigenesis that was attributed to the disruption of epithelial cell turnover and indicates that localized inflammation is critical for tissue repair. In contrast, the amelioration of colitis in mice lacking Nlrp6 and IL-1β production aligns instead with the destructive consequences of the inflammatory response. One intriguing reconciliation of these opposing findings may lie in the observation that NLRP6, by promoting ASC-dependent inflammasome assembly and IL-18 secretion, regulates the gut flora to maintain integrity of the epithelial barrier and reduce the incidence of colitis. , Therefore NLRP6 deficiency negatively regulates inflammasome assembly, promotes aberrant colonization of the gut by damage-inducing microbiota, and leads to a localized inflammatory response.
In contrast to what we believe to be the primary pro-inflammatory role of the other NLRs, NLRP6 instead may negatively regulate inflammatory signaling to retard pathogen clearance. Accordingly, mice lacking Nlrp6 are highly resistant to Listeria monocytogenes, Salmonella typhimurium, and E. coli that is attributed to NLRP6-mediated suppression of TLR-generated MAPK and NF-κB signaling events. Whether this finding is representative of a subclass of NLRs that function as critical immune suppressors remains to be determined. It does, however, provide tantalizing evidence of regulatory crosstalk between the NLRs and members of the TLR family.
The AIM2 Inflammasome
AIM2, although not an NLR, is a pyrin-containing protein, responsive to cytosolic DNA of either pathogen or host origin and able to mediate inflammasome formation ( Fig. 19.3D ). , AIM2, via its HIN-200 domain, directly associates with its trigger, DNA, to recruit the adapter ASC in a PyD-dependent manner to promote caspase-1 activation. ,
AIM2, by regulating the levels of IL-1β and IL-18, plays a critical role in antipathogen immunity to several cytosol-replicating bacteria such as Francisella tularenis , , and Listeria monocytogenes and the DNA viruses, vaccinia and cytomegalovirus. The mechanism(s) via which AIM2 can sense cytosolic DNA has yet to be fully elucidated, although type I interferon signaling events can contribute to the response to bacterial DNA although not to that of viral origin. ,
The ability of AIM2 to recognize and respond to cytosolic DNA of host origin is thought to mediate the pathophysiology of self-driven inflammatory responses including systemic lupus erythematosus and psoriasis.
Noncanonical Inflammasomes
The family of inflammatory caspases, in addition to caspase-1, includes murine caspase-11, and human caspases-4 and -5. Caspase-11 can induce pyroptosis in a manner that is independent of any known inflammasome. Several pathogenic bacteria, including E. coli, Vibrio cholera, Salmonella typhimurium, and Legionella pneumophila , induce the activation of procaspase-4, -5, -11. Expression of caspase-11 is induced by several pro-inflammatory stimuli including TLR4-TRIF activation via LPS, interferons IFNγ and IFNβ that is at least partially dependent on NF-κB and STAT signaling. Lipid A, a core structural moiety of lipopolysaccharide (LPS), was identified as the requisite trigger for caspase-4, caspase-5, and caspase-11 activation and pyroptosis following challenge with gram-negative bacteria. The activation of these caspases appears to occur by a direct interaction between LPS and the CARD of the caspase itself. The initiation of a process by the very same molecule responsible for its execution introduces an unexpected twist in our understanding of caspase biology and to the fundamental regulation of innate immunity. Whether this observation is only the first to define caspases as a novel class of receptors for pathogen-derived PAMPs remains to be seen.
Not all pyroptosis and IL-1β production is mediated by activation of caspase-1. In several studies, caspase-8, perhaps in a complex with MALT-1 and ASC, can mediate the processing of IL-1β and activation of Gasdermin-D to effect pyroptosis. It remains to be determined if this noncanonical caspase-8 inflammasome can be engaged in response to other extra-cellular pathogens, or, indeed, if the activity of other apical caspases can be similarly co-opted for host immune defense.
Molecular Pathways of Regulated Necrosis
Necrosis and Secondary Necrosis
Necrosis can be induced either directly by stimuli such as ATP depletion, nutrient withdrawal, and excessive ROS or, alternatively, as a consequence of apoptosis and is termed “secondary necrosis.” Cells undergoing secondary necrosis are characterized by chromatin condensation, a feature typically associated with apoptosis, and plasma membrane permeabilization, one of the hallmarks of necrosis. As noted previously, secondary necrosis can occur as a consequence of the cleavage of Gasdermin-E by executioner caspases, causing the formation of plasma membrane pores.
Necrosis and the Mitochondrial Permeability Transition
MOMP, most often a feature of apoptotic cell death, can also be associated with necrotic cell death arising from ischemia-reperfusion injury. Under these conditions, the loss of outer mitochondrial membrane integrity is attributed to activation of the permeability transition pore (PTP), a channel in the inner mitochondrial membrane, resulting in dissipation of the transmembrane potential, or mitochondrial permeability transition (MPT), swelling of the mitochondrial matrix, and, ultimately, rupture of the outer mitochondrial membrane and release of its contents into the cytosol. Importantly, however, there is no strong evidence to indicate that this activates apoptotic signaling, as would be the case if MOMP were mediated in a Bax/Bak-dependent manner.
PARP and Necrosis (Parthanatos)
The generation of ATP to provide the necessary energy to sustain normal cellular function is mediated by the collective activities of glycolysis, the Kreb’s cycle, and oxidative phosphorylation. Disruption of these pathways or an enhanced demand for intra-cellular energy consumption can lead to bioenergetic failure and engagement of necrosis. PARP is an essential enzyme for DNA repair, an energy-costly process that utilizes large amounts of nicotinamide adenine dinucleotide (NAD + ), thereby limiting mitochondrial ATP generation. As a consequence, cellular damage that demands excessive PARP-dependent DNA repair can disrupt oxidative phosphorylation, leading to necrotic cell death, also known as parthanatos (Thanatos—personification of death).
Ferroptosis
Ferroptosis or iron-dependent cell death is distinct from other forms of death by morphologic and metabolic criteria. Cells undergoing ferroptosis are characterized by mitochondria that are smaller in size but with an increase in the density of their membranes. The identification of several genes required for ferroptosis including citrate synthase, an acyl Co-A synthase family member (ACSF2) and an enzyme required for synthesis of poly-unsaturated fatty acids (ACSL4), both of which are involved in mitochondrial fatty acid metabolism, suggesting that lipid synthesis could represent one pathway through which ferroptosis may be regulated. Indeed, ferroptosis depends on the presence of poly-unsaturated fatty acids. Further characterization indicates that ferroptosis is induced by iron-dependent production of lipid peroxides via the Fenton reaction, dependent upon hydrogen peroxide (which in turn may depend on mitochondrial metabolism).
Peroxidized lipids are extremely toxic, as they promote a feedforward peroxidation in the presence of oxygen, destabilizing cellular membranes. The only lipid peroxidase in the cell, GPX4, is a seleno-protein required for the prevention of ferroptosis. GPX4 must be “recharged” by glutathione (GSH) to sustain its peroxidase activity. Therefore, inhibitors of GPX4 or treatments (or conditions) that deplete GSH (inhibition of cystine import via the System Xc − transporter, cysteine/cystine deprivation) promote ferroptosis.
Not all cells are sensitive to the induction of ferroptosis by GPX4 inhibition or GSH depletion. Remarkably, a wide variety of cancer cell lines that are relatively resistant to ferroptosis attain a dependence on GPX4 following treatment with a range of chemotherapeutic agents that kill a majority of the cells. Those that persist in the face of such treatment (“persister cells”) become highly susceptible to GPX4 inhibition. ,
Necroptosis
Until relatively recently, our understanding of necrosis was somewhat limited, fueled largely by the presumed absence of any underlying molecular mechanism. However, the identification of a form of regulated or programmed necrosis, necroptosis, has invigorated research efforts into determining the molecular basis for this type of death, how it contributes to host biology, and whether its regulatory pathways may be exploited for clinical benefit. ,
Although several stimuli can induce programmed necrosis, TNF and activation of its death receptor, TNFR1, has provided most insight into the molecular basis of this type of necrotic cell death (see Fig. 19.2C ). As we have already discussed, TNFR1 signaling is complex, with TNFR1-induced NF-κB activation promoting cellular survival and death receptor induced caspase-8 activation critical for the induction of apoptosis. However, long before we had defined its molecular basis or had a name for it, an alternative form of death receptor-induced death was described that proceeded independently of caspase activation and required RIPK1 activity. Recent genetic studies have characterized this form of TNF-induced caspase-independent cell death as necroptosis and defined key components of death receptor signaling, including caspase-8, FLIP L , and FADD as essential regulators.
An Unanticipated Finding of a Caspase Needed for Cell Survival
Tantalizing suggestions of an unidentified death pathway involving caspase-8, FADD, and FLIP arose from apparent contradictory findings that were not easily reconciled with their known role in apoptosis. These included the unexpected embryonic lethality associated with the deletion of caspase-8 or FADD, two proteins that prior to these studies were exclusively associated with promoting apoptotic cell death and therefore predicted to phenocopy the cellular accumulation observed in embryos lacking Apaf-1 or caspase-9. These findings were reconciled by the genetic rescue of the early lethality of caspase-8 or FADD deficiencies by the co-deletion of either of two related kinases, receptor-interacting serine/threonine-protein kinases (RIPK) 1 and 3. Programmed necrosis, or necroptosis, is therefore a death pathway that is mediated by RIPK1/3 but only in the absence of caspase-8 activity.
The overlapping requirement for several of the signaling components required for both TNF-induced apoptosis and TNF-induced necroptosis begs the question as to how the activity of caspase-8 is regulated under physiologic conditions to engage cell death via apoptosis or necroptosis, and even, under some circumstances, to sustain cellular survival.
Molecular Regulation of Programmed Necrosis
The realization that RIPK3 was required for TNF-induced RIPK1-dependent necrosis provided a critical piece of a mechanistic puzzle that was completed by the subsequent identification of MLKL as the target of RIPK3 and terminal effector of necroptosis. , Interaction between catalytically active RIPK1 and RIPK3 is mediated by their respective receptor interacting protein (RIP) homotypic interaction motifs (RHIM). , , Neither the homodimerization of the N termini of RIPK1 lacking a RHIM domain (RIPK1 ΔRHIM ) nor heterodimerization of RIPK1 ΔRHIM and RIPK3 ΔRHIM is able to trigger necroptosis. However, enforced dimerization of either the N termini of RIPK1 or RIPK1 ΔRHIM and RIPK3 are both able to do so, as are homo-oligomers, but not dimers of the C termini of RIPK3 ΔRHIM as well as dimers of the N termini of RIPK3 ΔRHIM .
RHIM-dependent interaction between RIP1 and RIP3 kinases triggers self-propagation of large hetero-oligomeric RIPK complexes that are essential for both kinase activity and execution of necroptosis. , , Characterization of these high order RIPK1/3 oligomers revealed strikingly similar characteristics to amyloid aggregates and an underlying mechanism by which kinase activation and necrosome assembly mutually reinforce each other. Amyloid-like structures can be toxic in and of themselves. However, acquisition of kinase activity as a consequence of RIPK1/3 complex assembly is more compatible with the requirement for specific RIPK substrates to mediate necroptosis, rather than the complexes themselves causing toxicity. Recently identified is the RIPK-dependent phosphorylation of the pseudokinase, MLKL, to induce its activation, oligomerization, and translocation to the plasma membrane. , , The swelling and loss of membrane permeability in cells dying by necroptosis is attributed to the ability of MLKL to disrupt ion homeostasis. Whether this occurs by the direct binding of MLKL to phospholipid components of the plasma membrane or by its association with Ca 2+ or sodium channels remains to be determined.
RIPK1—Apoptosis, Necroptosis, or Survival
While the activity of RIPK1 is critical for TNFR1-mediated activation of RIPK3 and execution of necroptosis, it is now apparent that its ability to regulate cell death mechanisms also encompasses roles in TNF-induced apoptosis under certain conditions as well as suppressing the aberrant inflammation associated with RIPK3 and caspase-8 activities. RIPK1 therefore clearly represents a critical signaling nexus within several pathways including those induced by TNF, TLRs, DNA-sensing pathways, and interferons. The diverse consequences of its regulatory activities are evidenced by its impact on multiple downstream signaling pathways and their biologic consequences.
Deletion of RIPK1 is associated with perinatal lethality characterized by multiorgan inflammation and widespread cell death. However, mice harboring one of several homozygous point mutations that eliminate RIPK1 kinase activity are viable. Recent genetic studies have begun to elucidate not only the molecular basis for the postnatal lethality associated with RIPK1 deletion but also the identity of signaling pathways regulated by RIPK1 and the mechanisms mediating its effects. While the individual deletion of FADD, RIPK3, or caspase-8 all fails to impact the lethal phenotype observed in RIPK1 −/− mice, the ablation of both RIPK3 and caspase-8 or RIPK3 and FADD in the context of RIPK1 −/− completely rescues postnatal lethality. Development also proceeds normally in mice lacking both RIPK1 and RIPK3 and the requisite adapter molecule for caspase-8 activation, FADD. The unambiguous conclusion from these studies is that RIPK1 is an inhibitor of two independent pathways associated with embryonic lethality—one that is mediated by FADD/caspase-8 and the other, a lethal RIPK3-mediated pathway. This is consistent with roles for RIPK1 in the inhibition of both apoptosis and necroptosis. But have we not just discussed the requirement for RIPK1 in promoting cell death? In fact, this assertion too is supported by unambiguous genetic data—specifically that the embryonic lethality associated with ablation of caspase-8 or FADD is completely rescued by the co-deletion of RIPK3 or RIPK1. , The simple conclusion, then, is that RIPK1 must drive or mediate the lethal signal resulting from the ablation of caspase-8 or FADD. Must we therefore reach the “not so simple” conclusion, then, that RIPK1 can both promote and inhibit cell death? As we will now discuss, that indeed is the case.
Homodimerization and activation of caspase-8 can be triggered by the ligation of TNFR1 via the adapter proteins TRADD and FADD to promote apoptosis. , , NF-κB, also activated by TNFR1 ligation, can inhibit caspase-8-dependent cell death by inducing transcription of FLIP, a catalytically inactive form of caspase-8. FLIP, by forming heterodimers with caspase-8, prevents the homodimerization of caspase-8 to inhibit apoptosis. RIP1K, by acting as a scaffold protein within this signaling complex, may provide a critical activation signal for NF-κB or facilitate amplification of NF-κB-mediated transcription. Either way, RIPK1 activity can contribute to cellular survival under conditions of TNFR1 ligation. Murine embryonic fibroblasts (MEFs) deficient for RIPK1, but not those expressing a kinase-dead version of RIPK1, are more sensitive to TNF-induced apoptosis. Furthermore, intestine-specific ablation of RIP1K in adult mice is associated with a postnatal lethality, characterized by extensive apoptosis of the gut epithelium and generalized inflammation. This RIPK1 null phenotype can be rescued by the tissue specific co-deletion of caspase-8 or FADD but not by the introduction of catalytically inactive RIPK1. , The observed accumulation of cIAP1 and TRAF2 in tissues lacking RIPK1 is consistent with a model in which the alleviation of cIAP1/TRAF2 mediated stabilization of NIK leads to increased NF-κB activity that promotes TNF transcription to induce apoptosis. This is supported by the rescue, albeit partial, of the excessive apoptosis associated with gut-specific ablation of RIPK1 as well as ripk1 −/− /ripk3 −/− -induced lethality, by the co-deletion of TNFR1.
RIPK1 can also mediate FADD-dependent caspase-8 activation to promote apoptosis in response to several stimuli including depletion of cIAPs, TNF, and TLR activation. Homotypic interaction between the DDs of FADD and RIPK1 to recruit and activate procaspase-8 constitutes the so-called “ripoptosome.” TLR-induced ripoptosome formation is mediated by interaction of the respective RHIM domains of TRIF and RIPK1. This prodeath role of RIPK1 is substantiated by several genetic studies in which the absence of RIPK1 (or caspase-8) can rescue the embryonic lethality associated with catalytically inactive RIPK3 D161N . In a similar manner, ablation of MLKL, the target of RIPK3 and required for necroptosis, instead can promote apoptosis via a RIPK3/RIPK1/caspase-8-dependent pathway.
The death-inducing role of RIPK1 is also evident in RIPK3/MLKL-dependent necroptosis, but only under circumstances in which caspase-8 activity is disrupted. RHIM-mediated interaction between the RIPK1 and RIPK3 is essential for RIP3K activity and the induction of necroptosis by ligation of TNFR1. For this, RIP1K absolutely requires its kinase activity, although the precise reason remains unclear, as RIPK1-mediated phosphorylation of itself, but not RIPK3 can be detected. Potentially, therefore, autophosphorylation and a consequent conformational change of RIPK1 may mediate its interaction with RIPK3, or alternatively, additional RIPK1 substrates that participate in the activation of RIPK3 to promote necroptosis remain to be identified.
RIPK1-Independent Necroptosis
While the ubiquitous activity of RIPK1 can be utilized for cellular survival, apoptosis, or programmed necrosis, RIPK3/MLKL-dependent necroptosis can also proceed in the absence of RIPK1 203 ( Fig. 19.4A ). TRIF, the signaling adapter associated with activation of TLRs 3 or 4, can directly bind and activate RIPK3 to promote necroptosis in the absence of RIPK1. TRIF-independent TLR signaling can also induce necroptosis by autocrine- or paracrine-mediated TNF activities. The DNA sensor, DAI, is activated by viral infection and contains an RHIM domain that can also directly engage RIPK3 function to promote necroptosis in the absence of RIPK1. Type I (α/β) and II (γ) interferons can also engage necroptosis under conditions in which either caspase-8 or FADD is inactivated. Although interferon-induced necroptosis is mediated via a RIPK1-RIPK3 complex, the kinase activity of RIPK1 is not required. Instead, the RNA-responsive kinase (PKR) interacts with RIPK1 to induce the formation of RIPK1-RIPK3 heterodimers and necroptosis. If RIPK1 is present under any of these circumstances, necroptosis can only proceed if the proapoptotic FADD-caspase-8-FLIP axis is disrupted.
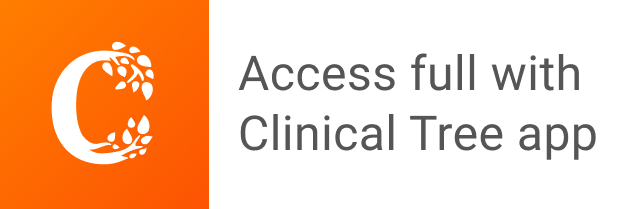