Healing is a complex process of orchestrated reactions and interactions with the goal of restoring structure and physical properties to damaged tissues. The musculoskeletal system is composed of different types of connective tissues. When healthy, each has a unique structure, function, and remodeling process. When damaged, they demonstrate unique healing processes. However, similarities in the process exist. Understanding these properties of healing is critical in the development and application of regenerative therapeutics. This article describes the common phases of healing, differences between healing in musculoskeletal tissues, factors that affect healing, and strategies to facilitate and optimize healing.
Key points
- •
When healthy, each type of musculoskeletal tissue has a unique structure, function, and remodeling process.
- •
When damaged, each type of musculoskeletal tissue demonstrates a unique process in healing.
- •
Understanding the unique properties of healing is critical in regenerative medicine, and in the development and application of regenerative therapeutics.
- •
There are many intrinsic and extrinsic factors that affect healing. An understanding of these factors can facilitate and optimize healing.
Introduction
Healing is a complex process of orchestrated reactions and interactions with the goal of restoring structure and physical properties to damaged tissues. The musculoskeletal system is composed of different types of connective tissues, including muscle, tendon, ligament, cartilage, and bone. When healthy, each type of tissue has a unique structure, function, and remodeling process. When damaged these tissues also demonstrate unique processes in healing. Despite unique features of repair in each tissue type, overarching similarities in the repair process also exist. Understanding these properties of healing is critical in regenerative medicine, and in the development and application of regenerative therapeutics. This article describes the common phases of healing, differences between healing in musculoskeletal tissues, factors that affect healing, and strategies to facilitate and optimize healing.
Introduction
Healing is a complex process of orchestrated reactions and interactions with the goal of restoring structure and physical properties to damaged tissues. The musculoskeletal system is composed of different types of connective tissues, including muscle, tendon, ligament, cartilage, and bone. When healthy, each type of tissue has a unique structure, function, and remodeling process. When damaged these tissues also demonstrate unique processes in healing. Despite unique features of repair in each tissue type, overarching similarities in the repair process also exist. Understanding these properties of healing is critical in regenerative medicine, and in the development and application of regenerative therapeutics. This article describes the common phases of healing, differences between healing in musculoskeletal tissues, factors that affect healing, and strategies to facilitate and optimize healing.
Phases of healing
Although each tissue type has unique aspects to healing, traditionally, healing has been divided into 3 common phases: inflammation, proliferation, and maturation.
Inflammation (from Injury Through Days 4 to 6)
Hemostasis
Hemostasis and inflammation begin immediately after injury and initiate healing. The body begins by controlling bleeding: injured blood vessels vasoconstrict and the coagulation cascade is activated. Platelets aggregate to form a clot made of collagen, thrombin, and fibronectin. The clot serves as a scaffold for invading cells and stimulates the release of cytokines and growth factors from the platelets, initiating the inflammatory pathway. Cytokines target hematopoietic cells to control immune response, and include chemokines, lymphokines, monokines, interleukins (ILs), colony-stimulating factors, and interferons. Growth factors act on nonhematopoietic cells to modulate healing, and include transforming growth factor (TGF), platelet-derived growth factor (PDGF), vascular endothelial growth factor (VEGF), epidermal growth factor, fibroblast growth factor (FGF), connective tissue growth factor, and insulin-like growth factor, among others. Overall, this phase results in vasodilatation and increased vascular permeability and migration of cells.
Inflammation
Inflammatory mediators modulate this phase of healing. Eicosanoids, a family of arachidonic acid (ArA) metabolites, including prostaglandins and leukotrienes, accumulate, drawing neutrophils into the injured area by IL-1, tumor necrosis factor (TNF)-α, platelet factor (PF)-4, and TGF-β. Neutrophils begin clearing cellular debris using proteolytic enzymes, including serine proteases and metalloproteinases, which digest collagen and break down the existing extracellular matrix (ECM) at the site of injury. Uninjured tissue is protected from these enzymes by protease inhibitors but may be overwhelmed by a robust inflammatory response.
Phagocytosis
Monocytes are attracted to the area by TGF-β, PDGF, PF4, IL-1, and leukotriene B4. Monocytes transform into macrophages around 48 to 96 hours after injury. The macrophages phagocytize the neutrophils and are stimulated by TNF and IL-1 to generate nitric oxide (NO), and additional matrix metalloproteinases (MMPs), clearing and allowing for cell migration into the ECM. Activated macrophages are critical in recruiting fibroblasts to the injured site, transitioning to the proliferative stage of healing. As the number of neutrophils and platelets increase, a cessation of the inflammatory phase of healing is triggered by lipoxins.
Proliferation (Day 4 Through 14)
Angiogenesis
The proliferative stage of healing is led by fibroblasts and epithelial cells. VEGF attracts endothelial cells to the injured area and initiates angiogenesis with the formation of new capillaries. The endothelial cells produce NO via endothelial NO synthase in response to hypoxia, causing vasodilation and increased blood flow to the site of injury.
Fibroplasia
Additional fibroblasts migrate to the injured site and are stimulated primarily by PDGF and TNF-α–derived from platelets and macrophages to begin synthesizing collagen, and a provisional matrix composed of type III collagen, glycosaminoglycans, and fibronectin. TGF-β further directs ECM production and a decrease in its degradation, stimulating fibroblasts to increase production of collagen.
Maturation and Remodeling (Day 8 to 1 Year)
The main feature of the maturation and remodeling phase is strengthening of the ECM and production of collagen in an organized network. Disruption of this stage may affect the strength and appearance of the repaired tissue. The preliminary ECM, composed of fibrin and fibronectin, glycosaminoglycans, proteoglycans, and other proteins, is replaced by an organized matrix made of stronger collagen fibrils. Over time, collagen deposition thickens and is organized along lines of stress. Even after maturation, the collagen in the repaired tissue will never become as organized or strong as the collagen found in uninjured tissues.
Musculoskeletal tissue types: differences in healing
To understand how each tissue type in the musculoskeletal system responds to injury, the basic structures and functions of each respective tissue must first be explored.
Muscle
Structure and function
Muscle is capable of contraction, which generates loads that are transmitted across joints to facilitate motion and provide stability. Myofibrils contain actin and myosin filaments, forming sarcomeres, the fundamental unit of muscle contraction. Myofibrils aggregate within the muscle cell to form fibers and are surrounded by endomysium. Fibers aggregate to form fascicles, encased by perimysium. Finally, fascicles aggregate and are surrounded by epimysium.
Healing
Repair of muscle tissue proceeds through the phases of inflammation, proliferation, and remodeling. When muscle tissue is injured, myofibers rupture along with local capillaries, a flux of calcium is released, and clot formation begins. Inflammatory cells migrate to the injured site, leading to phagocytosis of damaged tissues, and activation of fibroblast and satellite cells, which are myogenic stem cells. During the proliferative phase, satellite cells differentiate into myoblasts, fusing with the injured myofibers. In the remodeling phase, the new myofibers grow and differentiate into fully mature muscle fibers.
Tendon
Structure and function
Tendon is a mechanosensitive tissue that connects muscle to bone and transmits force to produce motion. Tendon attaches to muscle through the myotendinous junction and to bone through the fibrocartilaginous enthesis, rich in type II cartilage. Tendon has a hierarchical fibrillar structure. Triple-helical type I collagen molecules assemble densely into fibrils within an ECM, parallel to the muscle tendon axis, and along lines of stress. The ECM is composed of collagens, elastin, proteoglycans, and glycoproteins. Fibrils are then assembled into fibers, which are assembled into fascicles, surrounded by endotenon. Ultimately fascicles are bundled and surrounded by epitenon, forming the tendon unit.
Healing
Acute and chronic tendon injury may disrupt the highly organized structure of collagen in tendon. Most commonly, tendon injury results in tendinopathy but may also result in tendon tear. The pathophysiology of tendinopathy is not completely understood and is thought to result in part from impaired healing, with increased noncollagenous ECM, hypercellularity, and neovascularization. Additionally, the role of inflammation is not clearly established and is a point of controversy.
Tendon healing follows the phases of inflammation, proliferation, and remodeling. These tissues may heal at a slower rate than other connective tissues due to their dense and hypocellular nature. During the phases of tendon healing, tendon fibroblasts, or tenocytes, are the primary cell regulating homeostasis and respond to chemical and mechanical changes in the environment. These cells are responsible for collagen and other ECM matrix proteins during the proliferative stage of healing. Initially, these proteins are highly disorganized, primarily composed of type III collagen, and subsequently undergo remodeling during the final phase of healing, resulting in replacement with type I collagen, decreased cellularity and vascularity, and improved structure and strength. However, as with other connective tissues, healed tendon does not have the same mechanical properties of uninjured tissue.
Ligament
Structure and function
Ligament has a similar hierarchical structure to tendons, linking bone with bone, stabilizing joints, and generally operating under a reduced load compared with tendon. Predominantly, type I collagen is organized into parallel, crosslinked fibers along lines of stress. Ligaments function to provide passive joint stability through normal range of motion and to provide joint proprioception.
Healing
Ligaments are most often injured in traumatic joint injuries, resulting in either partial or complete discontinuity. Ligament healing proceeds through the 3 phases of inflammation, proliferation, and remodeling. Ligament healing depends on the size of the initial injury and whether contact exists between torn segments. During the first phase, retraction of the disrupted segments of ligament leads to gap formation that is filled with clot, which is subsequently resorbed and replaced with cellular infiltrate. Of note, several intra-articular ligaments, such as the anterior cruciate ligament, have limited clot formation at the site of injury, perhaps due to circulating levels of plasmin within the synovial fluid, which may prematurely dissolve the fibrin clot.
In the next phase, fibroblast proliferation increases production of collagen and ECM, bridging the torn ends of the ligament. Initially, this disorganized tissue is primarily composed of type III collagen. As healing proceeds into the remodeling phase, the healed tissue becomes more organized and collagen is arranged longitudinal to the multiple planes of force transmitted. Several changes persist after the conclusion of the healing cascade, including limited collagen crosslinking, increased cellularity, smaller collagen fibril size, increased vascularity, and abnormal innervation. Functionally, healed ligamentous tissue is less elastic than healthy tissue.
Bone
Structure and function
Bone provides structure and support for attaching soft tissues. Functionally, bone also serves as a home for hematopoiesis and calcium metabolism. Bone is a composite structure, including cells, ECM, and lipids. The fundamental unit of cortical bone is the osteon, a cylindrical structure, surrounded by several layers of lamellae, containing ECM and osteocytes. Lamellae are densely packed together and surrounded by a highly vascularized periosteum.
Healing
Bone also proceeds through the typical phases of inflammation, proliferation, and remodeling. The inflammatory phase of bone healing begins with bleeding from the periosteal vessels and clot formation at the site of fracture. Cytokines and growth factors are released and responsible for the migration and proliferation of chondroblasts and osteoblasts. The fracture gap is initially filled with granulation tissue and a primitive callus develops, providing some degree of stability at the fracture site.
During proliferation, the formation of callus continues, with the differentiation of chondrogenic stem cells into chondrocytes, producing cartilage to initially form of a soft callus. Osteoblasts gradually replace the cartilage in the soft callus with immature woven bone via endochondral bone formation, further stabilizing the injured site and forming a hard callus. The hard callus is further reinforced during the remodeling phase, where irregular woven bone is converted into lamellar bone, along lines of force, restoring strength and stability to the repaired bone. Remodeling may occur over months to years.
Cartilage
Structure and function
Articular, or hyaline cartilage lines the end of bones within joints. It can withstand tremendous forces generated through joints and allows for smooth gliding motion without significant friction. Articular cartilage is a highly organized, smooth, translucent tissue, which is hypocellular, aneural, avascular, alymphatic, and composed primarily of type II collagen, ground substance composed of proteoglycans, and elastin. The ECM of cartilage is hyperhydrated with water accounting for over 80% of the total weight. Chondrocytes are located within the ECM of the cartilage and are responsible for synthesis and maintenance of the ECM.
Anatomically, there are 4 main zones within articular cartilage that facilitate its attachment to subchondral bone. The superficial zone is the thinnest, with type II collagen oriented parallel to the articulating surface and with flattened chondrocytes. There is a random organization of type II collagen and spherical chondrocytes within the intermediate zone. The deep zone has type II collagen oriented perpendicular to the joint surface, with spherical chondrocytes. A tidemark then separates this basal layer from the final calcified cartilage zone that participated in endochondral ossification during growth.
Healing
The intrinsic capability of articular cartilage to repair is limited by the lack of vascularity and density of the ECM. The absence of blood supply inhibits the normal healing response that is present in the other connective tissues (see previous discussion). Chondrocytes are present in cartilage but trapped in lacunae and cannot easily migrate to damaged sites. Partial-thickness chondral defects may not show any healing response. On the other hand, a defect that penetrates the subchondral plate has a greater capacity to heal because it may facilitate clot formation and cell migration. However the resulting repair typically resembles fibrocartilage, rather than hyaline cartilage, with less stiffness and resilience.
Factors that affect healing
With an understanding of the phases of healing and the unique characteristics of each tissue type, one can appreciate the multiple factors that may alter the complex orchestrated process of repair and work toward optimizing the healing.
Local Factors
Oxygen
Healing requires energy in the form of adenosine triphosphate (ATP). Initially, anaerobic conditions produce ATP via glycolysis. As healing progresses, there is an increased need for energy and ATP is produced via oxidative phosphorylation and requires a rich blood supply. Limitation in oxygen available to the healing site may slow or halt healing. Fibroblasts are resistant to hypoxia and still proliferate in low oxygen environments. However, there is a marked decrease in collagen production in the presence of hypoxia. Hypoxia is also a powerful stimulus for angiogenesis and may explain the abnormal neovascularization seen in some forms of tendinopathy.
Hyperbaric oxygen therapy (HBOT) has been advocated as a treatment option for chronic wounds for many years, with the rationale that this may increase available oxygen at the site of wound healing. HBOT is not widely available and its use remains controversial. A systematic review has shown that HBOT decreases the rate of amputations in patients with diabetic foot ulcers but there does not seem to be a similar beneficial effect for other types of wounds, such as those due to venous stasis, arterial deficiency, or pressure. In musculoskeletal tissues specifically, HBOT does not affect recovery from delayed onset muscle soreness, and no clinical studies in humans have evaluated HBOT effects on tendon, ligament, and bone healing.
Pressure
Edema or elevated tissue pressures can prolong the inflammatory stage and delay the healing response. Mast cells produce numerous cytokines, histamines, and NO associated with increased inflammatory reaction, edema, and possible ischemia-reperfusion injury. A rise in internal or external pressure can increase capillary closure, thereby causing hypoxia and associated ischemic sequelae.
Mechanical
Normal development and remodeling of musculoskeletal connective tissues occurs with repetitive healthy loading. Mechanical loading is also essential in tissue response and repair to injury, particularly in the proliferation and remodeling phases because loads drive the anabolic response and reorganization of collagen and the ECM, as well as fluid exchange. Mechanical stimuli lead to a signaling response, involving growth and transcription factors; however, the precise involvement of these factors in development and repair is not completely understood. For instance, in tendon, tensile loads stretch tenocytes and activate protein kinases and an anabolic response, promoted by TGF-β, FGF, and transcription factors, including scleraxis, which is critical to tendon formation, differentiation, and modulating collagen synthesis.
Loading, rather than immobilization, may facilitate healing in tendon tissue. For example, loading stimulates repair of the Achilles tendon, which is impaired with immobilization. Immobilization in this case has been associated with decreased levels of ECM expression, loss of normal ECM structure, and altered tenocyte morphology, resulting in impaired function. Eccentric exercise therapy, with activation of the muscle and tendon while lengthening, has become a primary treatment for tendinopathy. These exercises improve symptoms and tendon structure. Optimal load, speed, number of cycles, and duration require further investigation for each tissue type. For instance, in the case of tendon healing, a 4% strain produced tenogenic differentiation, whereas an 8% strain initiated more adipogenic, chondrogenic, and osteogenic differentiation of tendon-derived stem cells. These different cellular responses to loading during healing may explain some features of failed healing in which tendons exhibit bone, fat, and cartilage tissue in areas of chronic injury. Tendon response to load is best understood in the context of a generalized stress-strain curve as shown in Fig. 1 . Using the correlation between tensile load and tendon deformation, it can be seen that various inflection points along the curve are areas for potential intervention. That is, an exercise program that could reliably produce 4% strain in a tendon would have potentially far-reaching clinical applications. However, in vivo measurement of tendon strain remains technically challenging. Newer imaging techniques, such as shear wave elastography, may offer guidance in these types of measurements in the future.
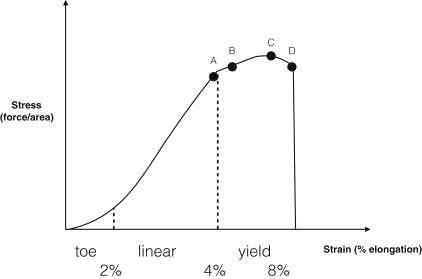
Bone healing is also affected by mechanical loading. Dynamic and cyclical, rather than static loading, stimulates proliferation of bone. Low-magnitude, high-frequency loads with periods of rest between loading periods have the greatest effect on healing. Additionally, compressive loading, rather than shear or tensile loading, produces increased mineralization and strength of callus formation. These observations form the basis for the use of low intensity ultrasound in bone healing. Ultrasound activates cell surface mechanoreceptors on osteoblasts, upregulating the production of growth factors and other proteins important in the healing response.
Systemic
Systemic conditions that affect healing have been studied in surgical patients to predict complications in reconstructive surgery. Though these factors have been described primarily in injuries to cutaneous and subcutaneous tissues, the same principles impact healing musculoskeletal injuries. These conditions may affect wound healing for a multitude of reasons. For instance, diabetes mellitus (DM) affects healing via vascular, metabolic, and neuropathic pathways. Conditions that significantly alter the immune system, resulting in increased immune response, inflammation, or immunosuppression can affect the healing. Additionally, age is associated with impaired healing; however, it is difficult to distinguish age alone from those of diseases commonly associated with increased age.
Perfusion
Decreased perfusion can be local (see previous discussion) or systemic due to cardiac dysfunction, peripheral vascular disease, or decreased circulatory volumes. Conditions that reduce systemic perfusion or decrease systemic oxygen concentrations will impair healing. Likewise, cardiovascular exercise can enhance perfusion and thus have a positive impact on healing.
Systemic diseases
Systemic diseases that alter immune function, protein metabolism, or collagen formation can impact tissue repair. Several examples include connective tissue disorders (CTDs), DM, hypothyroidism, malignancy, and organ failure. CTDs, such as Ehlers-Danlos syndrome or osteogenesis imperfecta, result in alterations in tissue strength, elasticity, integrity, and healing. Increased serum glucose levels in DM have significant deleterious effects on healing. The pathophysiology of impaired healing in DM was traditionally thought of as a microvascular occlusive process, though evidence supports additional mechanisms that impair healing, including increased levels of sorbitol, a toxic byproduct of glucose metabolism that may accumulate in tissues. Hypothyroidism is associated with decreased fibroblast function and collagen production, as well as systemic complications. Improved control of these conditions may result in improved healing response.
Malignancies can detrimentally affect healing for a multitude of reasons, including poor nutrition, organ compromise, immunosuppression, and hematologic effects; or as a result of therapeutics, such as antimetabolic, cytotoxic and steroidal agents, immunotherapy, and radiation. Chemotherapy results in bone marrow suppression and inhibits an appropriate inflammatory response and fibroblast collagen formation, whereas radiation increases free radical production, reduces tissue oxygenation, fibroblast proliferation, and inflammatory response.
Organ failure, whether gastrointestinal, renal, hepatic, or cardiopulmonary interferes with tissue repair. Gastrointestinal failure leads to disruption in absorption of critical nutrients and energy. Renal failure increases uremic toxins and a metabolic acidosis can affect healing by altering the immune system. Additionally, there are decreased neutrophil and lymphocyte responses in the setting of dialysis. Hepatic failure decreases clotting factors, protein production, and alters glucose regulation. Finally, failure of the cardiopulmonary system results in reduced perfusion or gas exchange and leads to hypoxemia and resultant tissue hypoxia at the site of injury.
Nutrition
Literature specifically evaluating the role of nutrition in the repair of the human musculoskeletal tissues is limited and current evidence is based primarily on animal models and research in surgical wound healing. Nutritional compounds play a critical role in protein synthesis and immune function, both integral to the healing process. The impact of nutrition on tissue repair can be divided into the correction of nutritional deficiencies and nutritional supplementation. Broadly speaking, there is good scientific support for correcting any nutritional deficiencies in the setting of healing but supranormal doses of micronutrients and vitamins do not seem to accelerate or improve a healing response.
Deficiencies of energy, macronutrients, and micronutrients can all impair healing. When metabolic requirements of healing exceed caloric intake, body fat and protein are consumed, particularly skeletal muscle. Insufficient nutrition can also elevate endogenous steroid levels, which is associated with muscle catabolism. Careful attention to energy intake is also needed. Following musculoskeletal injury, mobilization and activity levels are often reduced. Thus, one may conclude that energy requirements will decrease significantly following injury; however, this is not necessarily the case. Though prior activity levels will be reduced, tissue repair is an energy intensive process, with an increase of 15% to 50% depending on the type and severity of injury. Additionally, altered mobility may require increased energy expenditure. For example, ambulating with crutches may require 2 to 3 times the energy of normal ambulation.
Healing is heavily reliant on protein synthesis and the formation of collagen. Insufficient protein and amino acid intake will impair healing and increase inflammation. Arginine and methionine are important in collagen and matrix deposition, cellular proliferation, and angiogenesis. Supplementation with arginine has been shown to accelerate healing of pressure ulcers. Glutamine enhances the action of lymphocytes, macrophages, and neutrophils, whereas glycine plays a role in inhibiting leukocytes and is important in reducing inflammation-related tissue injury. High-quality or complete proteins found in animal products, meat, and soy contain all essential amino acids.
Oxandrolone, a synthetic analog of testosterone, has been studied in the setting of critical illness and burns, in which there is an increase in catabolism and decrease in protein reserve. In burn patients, the use of oxandrolone results in decreased nitrogen loss, lower loss of lean body mass, and shorter healing time for the donor site for skin grafts. Because musculoskeletal injury does not typically result in similar catabolic states, it is unclear if significant beneficial effects would be expected from similar treatments. The use of oxandrolone has not been formally evaluated in the healing of musculoskeletal injuries.
Omega-3 fatty acids have anti-inflammatory and immunomodulatory properties. Supplementation may be beneficial for inflammatory conditions or with excessive or prolonged inflammation. However, inflammation is a key phase of healing and careful consideration and use of anti-inflammatory nutrients is necessary because there is some evidence of impaired healing with supplementation of omega-3 fatty acids. Dietary fatty acids also play an important role in enhancing bone formation and suppressing bone resorption.
Micronutrients, including vitamins and minerals, are critical in immune function and healing ( Table 1 ). Trace metals are cofactors in collagen production. Deficiency is associated with impaired healing but there is no clear evidence to support supranormal intake during tissue repair. Vitamin C influences collagen modification, neutrophil function, acts as an antioxidant, and is the main vitamin associated with impaired healing. Supplementation with vitamin C has been shown to accelerate healing of pressure ulcers. It has also been shown to accelerate fracture healing in animal models. Vitamin A increases collagen synthesis, crosslinking, and inflammatory response. Importantly, vitamin A has been shown to reverse corticosteroid-induced inhibition of wound healing. High intake, however, may be linked to increased incidence of hip fractures and poor bone quality. Vitamin D is involved in controlling blood calcium levels, and appropriate levels of both substances are required for bone health and healing. Low vitamin D levels are also associated with delayed healing and reduced strength of rotator cuff tendons in animal models.
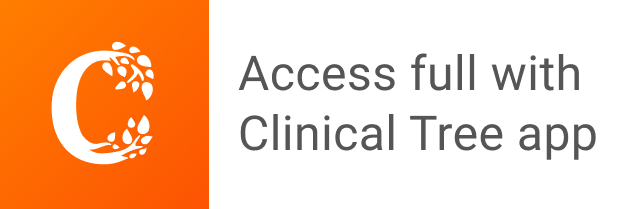