Skeletal Muscle Repair and Regeneration
Michael J. McClure, PhD
George J. Christ, PhD
Jonathan E. Isaacs, MD
Dr. McClure has received research or institutional support from MTF Biologics. Dr. Isaacs or an immediate family member is a member of a speakers’ bureau or has made paid presentations on behalf of Trimed; serves as an unpaid consultant to AxoGen, Inc.; has received research or institutional support from AxoGen, Cook Biotech, and Polyganics, Inc.; and serves as a board member, owner, officer, or committee member of the Hand Surgery Endowment Board of Governors. Neither Dr. Christ nor any immediate family member has received anything of value from or has stock or stock options held in a commercial company or institution related directly or indirectly to the subject of this chapter.
Keywords: atrophy; muscle injuries; regeneration; repair; skeletal muscle
INTRODUCTION
Skeletal muscle is highly organized and one of the largest organs in the body, comprising over 40% of total body mass. The primary function of skeletal muscle contraction is to enable motor tasks ranging from respiration to locomotion. The complexity of such a wide range of motor tasks is coordinated via neural control of both sensory and motor nerves, though it is the latter that are the focus of this report. In this scenario, multiple muscle fibers that belong to a given motor unit are innervated by a single motor neuron. Each motor unit is associated with one muscle fiber type pertaining to either slow twitch oxidative (type I), fast twitch fatigable (type IIb), or fast twitch fatigue resistant (type IIa). Thus, groups of motor units innervate a heterogeneous distribution of fiber types in each large muscle group and can vary depending on the activity and genetics of the individual. This distribution of motor units in association with innate genetics plays a key role in determining the endogenous regenerative capacity of skeletal muscle.1
At the cellular level, bundles of striated multinucleated myofibers derive their appearance from the characteristic longitudinal sarcomeric repeats, which, in turn, are surrounded by an intricate extracellular matrix and complex neurovascular networks. Although skeletal muscle has a relatively robust regenerative capacity, traumatic injuries and surgical excision, as well as a diversity of congenital and acquired disorders/diseases, result in simultaneous destruction and/or absence of muscle fibers as well as all the critical surrounding tissue components (nerve, vessel, matrix, and the muscle stem cell niche).2 Such injuries/conditions result in permanent functional and cosmetic deficits and are collectively referred to as volumetric muscle loss (VML) or VML-like injuries. By definition, the magnitude of tissue loss in VML and VML-like injuries exceeds the endogenous regenerative capacity of skeletal muscle.
The goal of this chapter is to illuminate the complex interplay among the vasculature, nervous system, and muscle satellite cells, and the resulting impact on muscle repair and regeneration in commonly occurring muscle disorders. To this end, we start with a brief review of normal muscle repair and regeneration, and then proceed to describe how this process is abrogated in various muscle conditions. Finally, we describe the current status and limitations of clinical care for muscle disorders, and moreover, highlight the potentially revolutionary impact that tissue engineering/regenerative medicine (TE/RM) technologies could have for improved functional outcomes.
SUCCESSFUL MUSCLE REPAIR AND REGENERATION
Skeletal muscle fibers are long multinucleated tubes with thousands of nuclei that form during embryogenesis and are maintained during postnatal development. These fibers are sensitive to mechanical, electrical, and chemical stimuli and respond rapidly following damage. In situations where the fibers are mildly damaged (such as during exercise), a process of repair and regeneration occurs via necrosis, inflammation, and regeneration. Furthermore, repair and regeneration are two distinguishable processes where we define repair as the preparation of an injured site through inflammation, cellular proliferation, and synthesis of new extracellular matrix to stabilize and preserve the tissue to form either newly regenerated tissue or scar. Regeneration is defined as the reestablishment of intact functional tissue that has histologic, biochemical, and mechanical properties similar to that of native skeletal muscle, following tissue repair. These processes are described in more detail below.
INFLAMMATION
The inflammatory response plays a critical role in muscle repair and regeneration after injury. Of the major constituents of the inflammatory response, macrophage participation is considered critical and is highly regulated. Successful regeneration relies on a delicate balance of phagocytic and nonphagocytic macrophages that secrete factors into the local microenvironment to activate regenerative pathways. For instance, acute injuries result in a predictable series of events in which neutrophils and macrophages play important roles in preparing the optimal local microenvironment for regeneration. More specifically, neutrophils release myeloperoxidase, inducing membrane damage that initiates macrophage phagocytosis and efferocytosis.3 This prompts the formation of distinct macrophage subpopulations that infiltrate the area to clear debris and promote regeneration. The first subpopulation to arrive is phagocytic CD68+ macrophages (M1), which clear debris and secrete pro-inflammatory cytokines such as interleukin-6 (IL-6). The second subpopulation of macrophages is a nonphagocytic CD163+/CD206+ phenotype (M2), which produces cytokines and growth factors regulating tissue regeneration. This shift in macrophage phenotype (polarity) is accompanied by increased release of pro-resolving and angiogenic milieu such as interleukin-10 (IL-10), vascular endothelial growth factor (VEGF), and transforming growth factor-β1 (TGF-β1) while simultaneously reducing pro-inflammatory signals like IL-6, IL-1β, and tumor necrosis factor-α (TNF-α).4,5,6
In this scenario, any imbalance may lead to impaired regeneration and favor fibrosis. For example, when the balance of phagocytic and nonphagocytic macrophages is disrupted, fibrosis can ensue through elevated numbers of M1 or M2 macrophages, shifting the scale in favor of one or the other. In fact, studies that ablated either M1 or M2 subpopulations demonstrated impaired regeneration by blocking removal of cell debris, or alternatively, blocking the release of growth factors and cytokines that promote angiogenesis and progenitor cell recruitment to the injury site.7
Muscle tissue pathology and abnormal repair and regeneration are closely linked to immune system regulation such as macrophage polarization following injury.8 Severe soft-tissue injuries caused by lacerations to the muscle belly, blast, crush, or tumor resection can alter this immune response cascade and create a microenvironment rich in TGF-β1 and IL-6 that permits fibrosis.9 In these types of injuries, the wound environment is mostly dictated by the first subpopulation of phagocytic M1 macrophages, while the second subpopulation of M2 macrophages are suppressed, limiting production of pro-regenerative factors and shifting the balance toward fibrosis.10 Furthermore, muscle atrophy caused by denervation or disuse upregulates signaling pathways such as TNF-α, which can have deleterious effects and permit aberrant synthesis of collagen.11 Lastly, prolonged muscular dystrophy causes enhanced fibrosis through continued breakdown and regeneration of muscle fibers, which leads to relentless M1 and M2 macrophage turnover and a slow buildup of fibrosis.9
REGENERATION
The generation of muscle fibers stems from a process termed, myogenesis, which occurs during both embryo development and adult regeneration in two phases, primary and secondary myogenesis. During primary myogenesis, mononucleated myogenic progenitor cells differentiate and fuse together to form long, syncytial multinucleated tubes. Multinucleated tubes grow in length and width during secondary myogenesis as more progenitor cells continue to fuse and myotubes fuse with each other. Three sources of muscle progenitors have been identified, including those that reside adjacent to myofibers (satellite cell), perivascular cells from the interstitial connective tissue, and some nonlocal cells derived from circulation such as mesenchymal stem cells.
Mesenchymal stem cells were shown to contribute to muscle regeneration following acute injuries, and specialized subpopulations that express PDGFR-a, CD34, and stem cell antigen-1 are considered to be more potent than other types.12 In particular, it was shown that these MSCs rapidly divide and then disappear following muscle injury, interacting with satellite cells to promote regeneration. In addition, it appears these cells boost the regenerative response by regulating inflammation through production of pro-inflammatory signals to recruit phagocytic macrophages during the first phase of inflammation followed by a change to anti-inflammatory factors to aid with regeneration.13
The role of perivascular cells (pericytes) has been studied in both prenatal and postnatal myogenesis. In postnatal tissue, these cells encircle the endothelium of microvessels, regulating blood vessel growth, permeability, and homeostasis. In muscle, two subpopulations were identified by their myogenic capacity where cells that stained for tissue nonspecific alkaline phosphatase and
CD146 possessed a stronger myogenic potential.14 Indeed, studies that extracted CD146+ cells from muscle reported positive expression muscle-specific transcription factors. More interestingly, a recent study demonstrated that prenatal pericytes directly contributed to the postnatal satellite cell pool.15
CD146 possessed a stronger myogenic potential.14 Indeed, studies that extracted CD146+ cells from muscle reported positive expression muscle-specific transcription factors. More interestingly, a recent study demonstrated that prenatal pericytes directly contributed to the postnatal satellite cell pool.15
The most widely accepted putative muscle progenitor cells are satellite cells. Satellite cells are found within the basal lamina of mature muscle fibers, reside as quiescent mononucleated cells within a specific niche, and are identified by their expression of Pax7, an important regulator of myogenic phenotype and cell maturation.16 Following mild injury or exercise, satellite cells are activated, exiting their quiescent state and entering mitosis to generate progeny that will go through several rounds of proliferation and then migrate to the injury site. During this proliferative state, a large percentage of daughter cells migrate, undergo differentiation, and fuse to form new myofibers. However, not all satellite cell progeny are involved in de novo fiber formation. In contrast, a smaller percentage of progeny will self-renew to replenish the muscle satellite stem cell population following full regeneration, entering back into quiescence.17 Although three cell types have been identified and discussed in brief, the focus of this chapter will emphasize the most prominently studied cell, the satellite cell.
SATELLITE CELL REGULATION
Satellite cells respond to environmental signals, including physical cues and regulatory factors that affect regeneration. This creates an extraordinarily complex microenvironment with diverse signaling patterns. Satellite cells are often located near capillaries, a source of endocrine signals, and are approximated to fibroblasts and immune cells, creating a source of paracrine signals which can influence satellite cell activation, proliferation, and differentiation. Neural inputs that depolarize the muscle fiber are also capable of influencing satellite cells through electrical activity, or by the chemical signals released following contraction or injury during exercise.18
Transforming growth factor-β (TGF-β) is a cytokine released by inflammatory cells following injury, stimulating resident fibroblasts to synthesize collagen and fibronectin. When levels of TGF-β are high, fibroblasts synthesize excessive amounts of extracellular matrix leading to fibrosis. This is observed in muscular dystrophy where dystrophic tissue has elevated levels of collagen compared with normal tissue. Furthermore, TGF-β promotes a fibrogenic phenotype in cultured myoblasts, indicating its dominant effect to promote fibrosis. In addition, recent evidence suggests that lower levels of TGF-β are required for effective regeneration, demonstrating a specific therapeutic window.19 In contrast, insulinlike growth factor-1 (IGF-1) is associated with increased muscle mass and enhanced regeneration in mice. IGF-1 functions in a dual manner, initially increasing proliferation in cultured myoblasts followed by enhanced differentiation and fusion.20 Hepatocyte growth factor (HGF) spikes in the early phase of regeneration. This protein is sequestered in extracellular matrix and released following injury, where it binds to the c-met receptor to activate satellite cells.21 Moreover, fibroblast growth factors (FGFs) were shown to induce myoblast proliferation and suppress differentiation. Like HGF, FGF-2 is sequestered in muscle ECM and promotes satellite cell proliferation during the early phase of regeneration.22
Aside from growth factor sequestration, ECM proteins play a critical role in regulation of satellite cell proliferation and differentiation. Satellite cells reside in a niche where they adhere to a basement membrane that contains a specific concentration of collagens, laminins, fibronectin, and proteoglycans. It is widely accepted that ECM influences satellite cell proliferation, migration, and differentiation.23 For instance, laminin and collagen are reciprocally regulated by fibronectin during muscle regeneration. In addition, fibronectin inhibits differentiation of cultured progenitor cells whereas laminin promotes it. Collectively, these diverse extracellular signals underscore the complexity of the satellite cell microenvironment and highlight factors that are capable of influencing muscle regeneration.
CONDITIONS WITH ALTERED MUSCLE REPAIR AND REGENERATION
As mentioned, skeletal muscle has the ability to fully regenerate following mild injuries by initiating a series of events. This ability is closely linked to the status of the muscle, where associated comorbidities should be considered as major determinants of regeneration. Changes muscle homeostasis (“status quo”) are capable of disrupting those regenerative and reparative processes by adversely affecting satellite cell pools via loss of neural control, disuse, trauma, or other dystrophic disorders (Figure 1). Below we provide some salient examples.
MUSCLE ATROPHY
Skeletal muscle is a highly adaptable tissue, changing its size, structure, and function in response to changing environmental stimuli. Part of this response involves a balance between catabolic (protein degradation) and anabolic (protein synthesis) pathways that either reduce or increase the size and mass of muscle fibers. When the catabolic pathways supersede anabolic, the balance shifts toward protein degradation and muscle atrophy. Atrophy occurs through multiple mechanisms including those induced by denervation, aging, and disuse. Although atrophy is not causing a direct injury to the muscle, it does impact satellite cell activity which can have detrimental effects on an individual’s ability to regenerate new muscle. Furthermore, each atrophic mechanism imposes a different effect on satellite cells.
DENERVATION ATROPHY
When muscle is denervated because of injury to the motor neurons through laceration, crush, or avulsion, the innervated muscle groups are paralyzed distal to the defect, creating a flaccid tissue with rapid atrophy and reduction in muscle mass
and function. Depending on the degree of denervation and length of time without neural stimulation (months or years), changes in muscle can be mild or severe. Short-term changes following denervation are characterized by progressive atrophy and functional deterioration (Figure 2). This can be observed at the muscle fiber level where ultrastructural changes become more prominent including disruptions of the Z-line and loss of actin and myosin filaments. Blood flow to muscles is also impacted with a reduced capillary bed density following the first week of denervation, compounding the effects of injury.24
and function. Depending on the degree of denervation and length of time without neural stimulation (months or years), changes in muscle can be mild or severe. Short-term changes following denervation are characterized by progressive atrophy and functional deterioration (Figure 2). This can be observed at the muscle fiber level where ultrastructural changes become more prominent including disruptions of the Z-line and loss of actin and myosin filaments. Blood flow to muscles is also impacted with a reduced capillary bed density following the first week of denervation, compounding the effects of injury.24
Denervated muscles are incapable of function without exogenous stimulation and, if spontaneous nerve regeneration does not occur, require surgery to reinnervate the muscle and reestablish neural connections.25 In cases involving a nerve laceration or avulsion, a proximal axonal stump remains, whereas the distal portion begins to degenerate. This pathological condition is known as Wallerian degeneration. Wallerian degeneration is initiated by the absence of a survival signal from cell bodies or a pro-degenerative signal at the injury site. Following loss of core survival signals like nicotinamide mononucleotide acetyltransferases (NMNATs), SARM1, and PHR1, axonal breakdown and fragmentation are activated.26 Furthermore, muscle and nerve attempt to preserve each other following injury and denervation. During the initiation phase of Wallerian degeneration, axons retain the ability to generate spontaneous miniature end plate potentials (MEPP) and end plate potentials (EPP) that can summate to evoke small muscle contractions. In addition, before full fragmentation, damaged axons release chemical factors that sensitize muscle to acetylcholine.24 Because muscle relies on neural stimulation to preserve muscle fiber integrity and vice versa, targets such as those mentioned could also help prevent the degenerative events that follow Wallerian degeneration in muscle. If reinnervation cannot be achieved within a certain time frame (˜18 months), the potential for recovery of muscle contraction and volume becomes less achievable.
Motor nerve injuries also cause changes in muscle fiber signaling patterns and membrane potentials. Following denervation, signaling favors the ubiquitin-proteasome pathway, where NF-κB activation causes release of several pro-inflammatory factors such as TNF-α and a variety of interleukins that collectively promote downstream pathways to degrade proteins.24 Activation of these pathways also causes increased production and localization of ion channels, hemichannels formed by connexins (39, 43, and 45), and pannexin channels via positive feedback, heightening membrane sensitivity to changes in electric potential, and producing a more permeable membrane for ion exchange.27 Altered sensitivity of muscle membranes via connexins increased the likelihood of continued atrophy. In support of this hypothesis a recent study demonstrated that absence of connexin 43 and 45 reduced the degree of atrophy,27 but more study is needed to determine whether targeting connexins is useful for denervation because these changes to the membrane occur in the short-term.
Increased abundance of channels in muscle membranes produced simultaneous increases in ion currents. Membrane depolarization is associated with changes in ion currents and membrane permeability. During the initial phase of denervation, an increase in intracellular Na+ and Ca2+ occurs alongside a reduction in K+. In addition, the sarcolemma becomes more permeable to Na+ and less permeable to K+, allowing for massive influx of Na+ to depolarize the membrane and create MEPPs to evoke miniature muscle contractions.28
SATELLITE CELL ACTIVATION FOLLOWING DENERVATION ATROPHY
Collectively, promotion of the ubiquitin-proteasome pathway and increased membrane permeability via gap junctions have a significant effect on satellite cell response. Increased inflammatory mediators and concomitant increased calcium flux activate satellite cell pools, sending progeny to repair damaged/denervated muscle. Satellite cell activity following short-term denervation increases dramatically with activation of the satellite cell pool and robust proliferation within the first 2 months (figure from our data). This is related to a general activation of the myogenic program following denervation with upregulation of early myogenic regulatory factors during
this early period. In contrast, long-term denervation encourages continued atrophy and degeneration of the original muscle fibers. Capillary loss becomes ever more prominent in the later stages of denervation with a 90% reduction 18 months postinjury.29 This occurs in conjunction with a replacement of muscle mass with noncontractile connective tissue. At this stage, there is no hope for recovery despite further efforts by satellite cells to regenerate new muscle fibers. Without innervated muscle and intact neuromuscular junctions, muscle deteriorates beyond repair.
this early period. In contrast, long-term denervation encourages continued atrophy and degeneration of the original muscle fibers. Capillary loss becomes ever more prominent in the later stages of denervation with a 90% reduction 18 months postinjury.29 This occurs in conjunction with a replacement of muscle mass with noncontractile connective tissue. At this stage, there is no hope for recovery despite further efforts by satellite cells to regenerate new muscle fibers. Without innervated muscle and intact neuromuscular junctions, muscle deteriorates beyond repair.
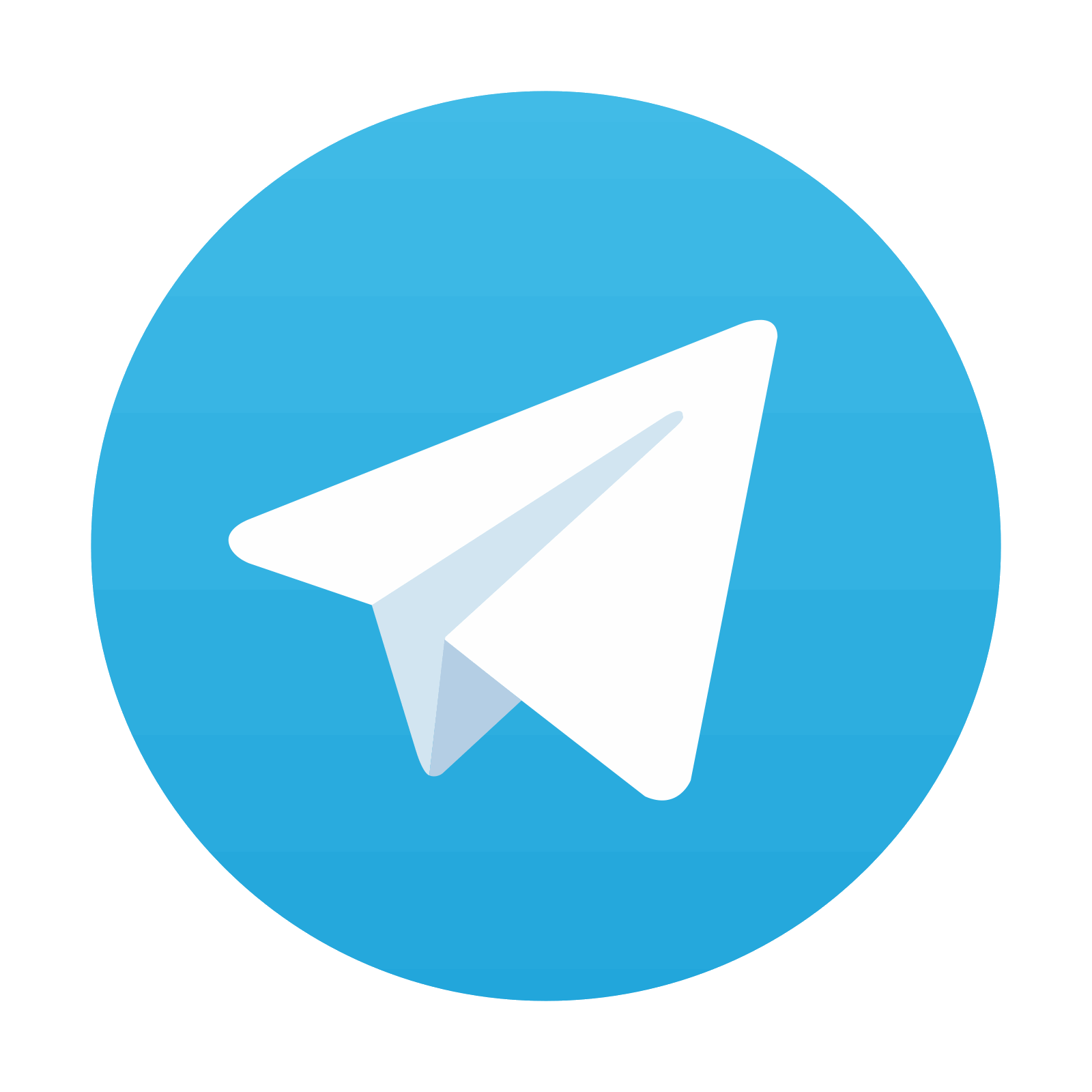
Stay updated, free articles. Join our Telegram channel
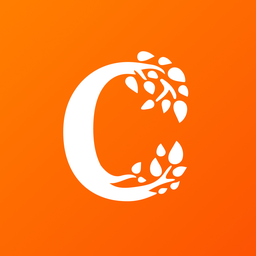
Full access? Get Clinical Tree
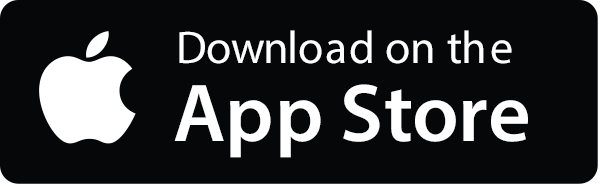
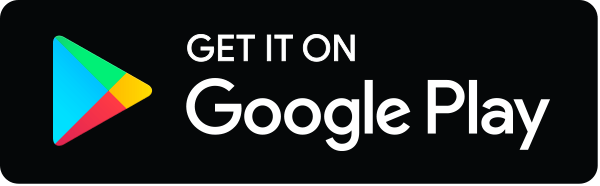