Pearls
- •
A wide selection of sedation and analgesia options is available in the pediatric intensive care unit.
- •
No ideal sedative agent exists for all patients.
- •
Most children do well with a combination of opiates (fentanyl or morphine) and supplementation with benzodiazepines either by infusion (midazolam) or on an as-needed basis (lorazepam) to provide adjunct anxiolysis and amnesia. Dexmedetomidine is being used increasingly as an adjunct in place of benzodiazepines.
- •
Postoperatively, patients require adequate analgesia, which may include the use of epidural anesthesia or patient-controlled analgesia.
- •
When the ability to perform a rapid neurologic examination is necessary, use of short-acting agents—such as remifentanil, propofol, or isoflurane—may be warranted.
- •
All sedative agents result in tolerance with prolonged use. The clinician must be aware that withdrawal may occur with the prolonged use of these agents (i.e., more than 3–5 days).
- •
A proactive treatment plan with methadone at the equivalent dose can effectively and safely prevent opiate withdrawal in the patient in the pediatric intensive care unit.
Sedation is an integral part of patient management in the pediatric intensive care unit (PICU). It is necessary to minimize the perception of and response to anxiety and pain. Children who are not adequately sedated or who experience pain may become tachycardic and hypertensive, among other signs of stress. Provision of adequate sedation and analgesia facilitates bedside nursing, rehabilitation, and respiratory care. It also prevents dislodging of critical tubes and monitoring devices, such as central venous lines, arterial lines, chest tubes, and endotracheal tubes. Optimizing sedation and analgesia has been shown to reduce oxygen consumption in critically ill children and modulate the intensity of stress inflammatory response. Conversely, oversedation can cause cardiovascular depression, ileus, interfere with a comprehensive neurologic examination, and prolong the ICU stay. In patients requiring prolonged sedation, tolerance and tachyphylaxis develop, which lead to increasing sedative requirements. The patient may also be at risk for postsedation-related complications, such as delirium and post-ICU psychological disorders.
There are different sedative classes available to use in the PICU. It is worth noting that the majority of these agents are not approved by the US Food and Drug Administration (FDA) for long-term sedation in children and that most of the experience with these medications comes from short-term use in the operating room. Their effectiveness, safety, and adverse effect profiles may not transfer between these two environments. The possibility of impaired brain development after anesthesia in young children must also be a concern for the PICU physician.
Patients may recall their stay in the ICU despite adequate sedation. Many remember having an endotracheal tube or having their lungs mechanically ventilated. Nightmares and hallucinations also have been reported. Single-drug therapy or inadequate dosing may be associated with a heightened incidence of recall in the patient receiving neuromuscular blocking agents. In adults, delusional memories and underlying anxiety states may predict the development of posttraumatic stress disorders after sedation in the ICU. Delusional memories were reported much more frequently than factual memories, and most patients did not remember correctly the events that occurred during their stay in the ICU. In pediatric patients, recall of the PICU experience has also been reported. More than 66% of pediatric patients remembered their stay in the PICU; 18% had bad memories, 16% remembered mechanical ventilation and anxiety, and 29% remembered pain from a procedure or movement. Overall, approximately 15% of the recollections among PICU patients were considered negative. Verbal children with normal cognition have had delusional memories, often reported as disturbing visual hallucinations. Delusional memories were more common with longer use of opiates and benzodiazepines, which is consistent with the adult literature. Children with head injuries are less likely to report factual memories. Sleep disturbance and loss of sleep are reported to adversely affect short-term recovery and long-term cognitive function. A normal sleep-wake pattern has benefits with respect to immunity, thermoregulation, reducing the risk of delirium, and preventing the development of a catabolic state. The sleep that children experience under sedation is not restful, with decreased slow-wave and rapid eye movement sleep patterns and loss of circadian rhythm. Noise levels in the PICU are often high and can interfere with the child’s ability to rest. Monitor alarms and awakening during routine assessments contribute to this distraction. The use of earplugs and eye masks may help reduce the disruption and facilitate a more normal sleep pattern.
Posttraumatic stress disorder
Posttraumatic stress disorder (PTSD) is a possible complication of a child’s stay in the ICU. Several components are required for the diagnosis of PTSD. The American Psychiatric Association’s Diagnostic and Statistical Manual, Fifth Edition (DSM-V) requires the following criteria for a diagnosis of PTSD in adults, adolescents, and children older than 6 years: (1) an exposure, (2) one or more intrusion or reexperiencing symptoms, (3) avoidance behavior, and (4) alterations in arousal associated with the traumatic event; in addition, (5) the symptoms should have exceeded 1 month and (6) have a clinically significant effect on the child’s functioning. There is no specific test for PTSD, but children often have a low basal cortisol level, with exaggerated cortisol suppression in response to dexamethasone.
Using slightly modified alternate DSM-V criteria shown to be more appropriate for children ( Box 132.1 ), the incidence of post-PICU PTSD was 29% in children aged 6 through 16 years. There appeared to be no difference in the incidence with respect to age; however, younger children tended to have more avoidance of thoughts, whereas older children suffered from diminished interest in activities and difficulty concentrating. This is important, as these findings may persist for up to 6 months and affect the daily function of the child and family after discharge. Children who are more critically ill or those who require multiple procedures are more likely to report symptoms of PTSD. High post-ICU anxiety scores and delusional memories (rather than factual ones) appear to be related to higher risk of PTSD. ,
Criterion A (event)
Experienced event that threatened death or serious injury
Criterion B (reexperiencing)
Recurrent distressing memories
Recurrent distressing dreams
Sense of reliving events
Psychologic distress with reminders
Physiologic reactivity with reminders
Criterion C (avoidance)
Avoidance of thoughts, feelings
Avoidance of activities
Diminished interest in activities
Detached from others
Restricted affect
Sense of foreshortened future
Criterion D (hyperarousal)
Difficulty sleeping
Irritability/anger
Difficulty concentrating
Hypervigilance
Exaggerated startle response
Criterion E (duration)
Duration greater than 1 month
Criterion F (effect)
The disturbance causes clinically significant distress or impairment in social, occupational, or other important areas of functioning.
The use of dexmedetomidine has been shown to reduce the risk of delirium; however, it is associated with increased recall along with greater post-ICU anxiety. It is important to ensure that children are suitably sedated and receiving appropriate analgesia to reduce the unpleasant memories of painful procedures during their ICU stay.
Sedation scoring and assessment
Various scoring systems have been used to guide sedation. A recently validated and now often used assessment tool is the Richmond Agitation Sedation Scale (RASS). The RASS grades a patient’s level of sedation using a 10-point score ( Table 132.1 ) and appears to be better suited for PICU sedation than the Ramsay score. Many units use protocols in which the bedside nurse assesses the patient’s RASS score and then adjusts the sedative medication dosing as necessary to achieve the desired level of sedation, which varies from patient to patient and over time. Most clinicians seek to maintain instrumented patients in a sleepy but easily awakened state. A RASS score of −2 to −3 seems to be the ideal clinical end point for sedation for most patients. Deeper sedation (RASS −4) should be reserved for selected patients who are often younger, are receiving concurrent neuromuscular blockade, or have severe head injury. The use of sedation scoring systems has been shown to reduce costs in surgical ICUs. It allows patients to be weaned more rapidly from the ventilator through better control of the sedation level, resulting in more ventilator-free days and shorter ICU stay. The COMFORT scale, which is composed of eight variables (each with five categories), has also been validated for use in the PICU to assess sedation level in children. However, use of this system is more complicated and time consuming.
+4 | Combative | Unacceptable; increase sedation |
+3 | Very agitated | Unacceptable; increase sedation |
+2 | Agitated | Unacceptable; increase sedation |
+1 | Restless | Acceptable; no action necessary |
0 | Alert and calm | Acceptable; no action necessary |
−1 | Drowsy | Acceptable; no action necessary |
−2 | Light sedation | Acceptable; no action necessary |
−3 | Moderate sedation | Acceptable; no action necessary |
−4 | Heavy sedation | Unacceptable; monitor respiratory status and sedation level until stable at 2 or 3 |
−5 | Unarousable | Unacceptable; monitor respiratory status and sedation level until stable at 2 or 3 |
Clinical scoring systems may be affected by some degree of subjectivity and interobserver variability; thus, more objective assessment of sedation could be beneficial. The Bispectral Index (BIS) is a processed electroencephalogram (EEG) monitor that measures the hypnotic effects of anesthetics and sedatives. It is an empirical, statistically derived measurement that has been validated as a measure of hypnosis in adults in the operating room and ICU as well as in children in the PICU. It does not measure the concentration of a particular drug. The BIS monitor reports a single number from 0 to 100 that represents an integrated measure of cerebral electrical activity. A number of 100 on the BIS score indicates that the patient is fully awake, whereas a number less than 40 suggests a deep hypnotic effect. A BIS value of less than 60 in surgical patients was not associated with a recall of intraoperative events. The use of the BIS monitor in adult surgical patients and in older pediatric patients has been shown to reduce anesthesia requirements and result in a shorter recovery time. In critically ill adults, the BIS score has shown good correlation with various sedation scores.
One of the main shortcomings of clinical sedation scoring systems is their inability to assess depth of sedation in patients receiving neuromuscular blocking agents (NMBAs). Patients who receive NMBAs in the operating room or in the PICU are at increased risk of awareness during anesthesia. Clinical signs may not reliably assess adequacy of anesthesia or sedation in the PICU patient since alterations in heart rate, blood pressure, perfusion, and pupillary responses can occur due a variety of other reasons. A study using BIS monitoring in pediatric patients receiving NMBAs found that the PICU bedside nurse’s assessment detected only 8% of patients at risk for awareness and recall (BIS ≤80; Fig. 132.1 ).

An important aspect of sedation monitoring is the level of stimulus. The BIS monitor will estimate the degree of hypnosis at a point in time in the context of the balance between sedation, analgesia, and stimulation. It cannot predict what will happen if this balance is changed. Although the BIS monitor is used in many institutions, the question of whether its use can prevent awareness under anesthesia is still being debated. A study of 2000 adults whose anesthesia was titrated to a BIS score of lower than 60 or by the end-tidal inhalational concentration of the anesthetic agent to at least 0.7 minimum alveolar concentration (MAC) found two cases of awareness in each group. The BIS score was greater than 60 in one case of awareness. Although the BIS monitor was not able to reduce this low incidence of awareness, the level of anesthesia between the groups was very similar. This study was severely underpowered to show any benefit. However, the combination of end-tidal monitoring and BIS monitoring may help to reduce intraoperative awareness.
Another potential concern with using the BIS monitor is its relevance or reliability for the pediatric patient. Research thus far does not show a correlation with the myriad of sedation scoring systems used in the PICU. Furthermore, the lack of continuous sedation assessment by these scoring systems, as is possible with BIS monitoring, makes comparison difficult. It also is unclear whether the EEG analysis algorithms used in the BIS monitor are applicable to the pediatric brain. This issue needs further evaluation before BIS monitoring can be considered standard in the PICU. Pediatric-specific BIS probes are now available; its use for patients 1 year and older appears to be appropriate. The possible inaccuracy of the BIS in younger children has been demonstrated in the operating room, where BIS values in younger children (age 1–12 years) tended to be higher (>60) than in older children receiving the same propofol, remifentanil-based anesthetic. In this study, there was no recovery benefit to a BIS-driven anesthetic compared with a standard dose-based, clinical signs–driven anesthetic. In fact, the younger children took longer to wake up due to high doses of sedation agents received in an effort to keep the BIS score less than 60. Children with the BIS greater than 60 appeared to be appropriately anesthetized; thus, the concept that the BIS score must be less than 60 to ensure adequate anesthesia in younger children may not be correct. This may correlate with the need to select a different BIS end point in the PICU. In a recent study in the neonatal population, the BIS score correlated well with deep sedation but was unable to differentiate between no sedation and light sedation. This may be related to a generally slower background cerebral activity in neonates as compared with older children and adults.
Finally, the BIS monitor is less reliable when used with certain hypnotic agents, such as ketamine, dexmedetomidine, nitrous oxide, xenon, and opiates. Its accuracy is decreased by patient movement, the use of high-frequency oscillatory ventilation, underlying seizures, or any form of brain injury.
Other processed EEG sedation assessment monitors are now available. The SNAP II monitor uses a different spectrum of EEG frequency analysis, but little has been reported with the SNAP II monitoring in pediatric patients. In the PICU, SNAP II was found not to correlate well (r = 0.18) with the sedation depth of intubated children. The mean SNAP index changed only between 58 and 68 over a sedation score reflecting deep to light sedation.
Opioids and analgesia in the pediatric intensive care unit
Sedation in the PICU is most commonly achieved with a mixture of opioids and benzodiazepines (BZDs). Although many synthetic and naturally occurring opioids exist, morphine is considered the agent against which others are compared. The primary source of morphine is opium obtained from the opium poppy (Papaver somniferum), which also produces alkaloids such as codeine, thebaine, papaverine, and noscapine. Opiates are substances derived from opium; the term opioid also describes substances derived from opiates (e.g., oxycodone) but includes substances that are created synthetically and have properties that are similar to those of opiates (e.g., fentanyl and methadone). The terms often are used interchangeably because the pharmacologic effects fall into the same category. Opioids are agonists at various opioid receptors, for which several endogenous ligands exist. There are three major classes of receptors: mu (μ), kappa (κ), and delta (δ; Table 132.2 ). Opioid receptors possess the same general structure: an extracellular N-terminal region, seven transmembrane domains, and an intracellular C-terminal tail structure. Subtypes of each receptor (e.g., μ 1 , μ 2 ) exist, as do less well-characterized opioid receptors, such as nociceptin.
Receptor | Endogenous Ligand | Agonist | Antagonists |
---|---|---|---|
μ | β-Endorphin | Morphine | Naloxone |
Endomorphin | Fentanyl | Naltrexone | |
δ | Leu-enkephalin | DPDPE | Naloxone |
Met-enkephalin | Naltrindole | ||
κ | Dynorphin | Buprenorphine | Naloxone |
Nociceptin | — | — |
Most of the therapeutic and adverse effects can be accounted for by agonist activity at the μ-receptor, which is responsible for analgesia, respiratory depression, pupillary constriction, and euphoria. At the cellular level, μ-receptor activation through a coupled G protein inhibits adenylate cyclase, resulting in inhibition of voltage-gated calcium entry channels and activation of potassium channels. This results in a loss of potassium from the neuron, causing hyperpolarization and depression of excitability in the nervous system. Associated effects on cholinergic, adrenergic, serotonergic, and dopaminergic neurotransmitter systems are seen within the central nervous system (CNS). These receptors are found at multiple sites along pain pathways, including the spinal cord, midbrain, thalamus, and cortex. At the spinal cord level, pain reflexes (nociceptive) are depressed by receptors in the substantia gelatinosa, which are mostly presynaptic and inhibit the release of substance P from C-fiber nerve terminals and account for the effectiveness of opioids administered intrathecally and epidurally. In the midbrain, the analgesic effect is mediated in the periaqueductal gray matter through ascending fibers and descending fibers that modulate the function of the dorsal horn. Acetylcholine, γ-aminobutyric acid (GABA), norepinephrine, and serotonin also are involved in these pain-modulating pathways. Peripheral opioid receptors also exist and can be expressed in response to inflammation. The intraarticular injection of morphine produces analgesia following arthroscopy through activation of opioid receptors located on white blood cells.
The endogenous ligands for the opioid receptors are the enkephalins, endorphins, and dynorphins. They have a morphine-like effect that can be specifically antagonized by the μ-receptor antagonist naloxone. The endomorphins have potent analgesic and gastrointestinal (GI) effects. At the cellular level, they activate G proteins ([35S] GTP γ-S binding) and inhibit calcium currents. Pro-opiomelanocortin is the precursor for β-endorphin (as well as adrenocorticotropic hormone and melanocyte-stimulating hormone). β-Endorphin, itself very active, also includes the amino acid sequence for met-enkephalin. The main precursor is proenkephalin A, which contains four copies of met-enkephalin and one copy of leu-enkephalin. The met-enkephalin sequence also gives opioid activity to a number of other larger peptides. Proenkephalin B (prodynorphin) gives rise to the dynorphin series and contains three leu-enkephalin sequences. Local application of these endogenous substances to the brain provides effects that are similar to those of opiates. They arguably do not function as analgesics in basal states because the administration of naloxone does not cause pain in the normal state. They are released during periods of sustained pain, stress, or activity to modulate physiologic pathways, including those involved with pain. Therefore, they are probably important to the physiologic condition of the patient in the ICU.
Specific opioid agonists
Morphine
Morphine is an opiate, its primary therapeutic actions being sedation and analgesia; anxiolysis and euphoria also may occur. These four therapeutic effects may be exploited to the benefit of the patient. These actions are mediated through the periaqueductal gray matter, ventromedial medulla, and spinal reduction of nociceptive reflexes occurs all over the body, even below a completely transected spinal cord. In addition to increasing the sensory threshold for pain, morphine may decrease the hurting aspect (or unpleasantness) of pain. A patient given morphine may say something such as “I have just as much pain, but it doesn’t distress me as much.” It blunts most types and intensities of pain, although some forms of neuropathic pain are relatively resistant. The resulting analgesia may be potent enough to abolish diagnostic symptoms and signs. The sedative effects reduce higher cortical function, cause difficulty in concentration, and cause a sense of drowsiness and dream-filled sleep. Higher doses will cause a state of unconsciousness or coma. The rate of respiration is reduced, with a resultant fall in minute ventilation despite an accompanying increase in depth of breathing. This effect is associated with a decreased responsiveness to carbon dioxide (CO 2 ) and is additive to the decreased CO 2 response seen during sleep. In some circumstances, respiratory drive may be limited to hypoxic stimulation of the carotid chemoreceptors; this is the most serious dose-related adverse effect of morphine. It can occur at doses used clinically for analgesia. In general, all opiates produce the same degree of respiratory depression when given in equipotent doses and for any given level of analgesia. Opioids do not have anticonvulsant properties, whereas meperidine (and its metabolite normeperidine) may lower the seizure threshold.
Another CNS effect of morphine is pupillary constriction due to a central effect on the oculomotor nucleus. Nausea results from stimulation of the chemotrigger zone. However, opioids also depress the vomiting center; thus, the final effect is unpredictable. Nausea and vomiting are much more frequent in ambulatory patients than in patients confined to a hospital bed. Morphine can modify stress-related endocrine responses. It decreases the release of several hormones, including adrenocorticotropic hormone, antidiuretic hormone, prolactin, growth hormone, and epinephrine. The neuroendocrine stress response that is normally seen with trauma and surgery may be blunted. Itching may be caused by histamine release, but it also may be due to opiate receptor activation in the spinal cord.
Morphine’s effects on smooth muscle cause constipation. It reduces the intestinal propulsion activity through its central and peripheral effects. The central effects may be mediated by the vagus nerve. The direct smooth muscle relaxation and the increased local cholinergic transmission can be partly reversed by naloxone. This decreased motility is the basis of several over-the-counter antidiarrheal preparations, including diphenoxylate, a μ-agonist that does not cross the blood-brain barrier and thus acts as a peripheral opioid agonist. Morphine causes an increase in biliary tract tone, which may cause biliary colic as well as increased tone in the bladder detrusor muscle and vesical sphincter. Urinary retention is common with opioids, occurring in 55% of children receiving spinally administered opioid and 20% receiving intravenous (IV) opioid.
Morphine has been studied extensively in term and preterm neonates. Glucuronidation is present in term babies and in many preterm ones. The half-life of morphine, however, is 2 hours in children, 6.5 hours in term neonates, and 9 hours in the preterm child because of reduced clearance. Volume of distribution does not vary with age. Morphine causes histamine release and can cause peripheral vasodilation. Infused at analgesic doses, it has little effect on the cardiovascular system, but skin flushing is not uncommon with rapid IV administration. The histamine-releasing potential should be considered in patients with asthma, especially during an acute exacerbation, and in patients with unstable cardiovascular systems for whom safer alternatives exist, such as fentanyl.
Dosing recommendations in the ICU include a bolus dose of 0.05 to 0.10 mg/kg and an infusion of 10 to 50 μg/kg per hour. Fifty percent of these doses should be used if the patient is younger than 3 months of age. All opiates are weak bases and are moderately ionized at pH 7.4. Oral morphine is effective but undergoes hepatic first-pass metabolism, which is variable among patients. The oral dose for acute pain is 2 to 5 times the IV dose, whereas in long-term use the oral dose is 1.5 to 2.5 times the IV dose.
Morphine is metabolized to morphine-3-glucuronide (M3G) and M6G in the liver. M3G is the major metabolite and has little morphine-like activity, although there is some suggestion that M3G may be associated with an antinociceptive effect, accounting for failure of analgesia during long-term use. In contrast, M6G is many times more potent than morphine itself. Morphine undergoes significant first-pass hepatic metabolism, whereby after a single parenteral dose, only morphine is initially active. After a single dose by mouth (PO), both morphine and M6G are active. With long-term oral use, M6G accumulates until its analgesic effect is greater than that of morphine. A similar effect can be anticipated with long-term morphine infusion in the patient in the PICU. The kidney excretes the glucuronides, together with only a small amount of free morphine. Ninety percent of total urinary excretion occurs within 24 hours.
Tolerance , defined as an increase in the dose required to create the same response, is a potential problem with all opiates. Tolerance is mainly limited to the depressant actions of morphine, including analgesia, respiratory depression, anxiolysis, and drowsiness. Tolerance of morphine’s inhibition of bowel motility and pupillary constriction is minimal. The mechanism of tolerance appears to involve the degree and duration of both μ- and κ-receptor occupancy. It appears more rapidly after continuous infusion, and cross-tolerance to other opiates is common, although anecdotal evidence suggests that a dose reduction may be possible when opioids are switched because cross-tolerance sometimes appears incomplete. Receptor downregulation also may occur as well as altered metabolism with an increased M3G/M6G ratio. Simultaneous blockade of N- methyl- d -aspartate receptors is effective in reducing the development of tolerance. Clinical tolerance appears uncommon with an exposure of less than 3 days. However, after prolonged administration, doses 10 to 20 times that which would cause respiratory arrest in naïve patients may be tolerated.
Meperidine
Meperidine has one-tenth the potency of morphine. Compared with other common opioids, meperidine has more CNS excitatory effects, including tremors, muscle spasm, myoclonus, psychiatric changes, and seizures. These effects may be due to a central serotoninergic effect. It is metabolized by the liver to normeperidine, which is twice as toxic as meperidine and has a longer half-life (15 hours). Normeperidine accumulation is enhanced in patients with an induced cytochrome P450 system. Meperidine has a shorter duration of action (2–3 hours) and has a more rapid onset because of its increased lipid solubility compared with morphine. Meperidine is unique among opioids because of its local anesthetic properties, which are capable of providing surgical spinal analgesia. A small dose (0.125–0.250 mg/kg) of meperidine may be used to treat postoperative shivering.
Fentanyl
Fentanyl is one of the most commonly used opiates in the ICU. It is a synthetic derivative of meperidine without many of its unwanted side effects. It is a potent μ-agonist and is 100 times more potent than morphine. It has a rapid onset and cessation because of its high lipid solubility ( Fig. 132.2 ). Fentanyl may be administered by several routes, including IV, intramuscular (IM), transmucosal, and subcutaneous (SC) when venous access is inadequate. Fentanyl may be given intranasally as an alternative to the transmucosal route. It has been used for premedication, sedation, and for the treatment of hypercyanotic spells in tetralogy of Fallot. There is also a 12.5-μg transdermal patch available for children designed to release 12.5 μg/h of fentanyl for approximately 3 days. It is indicated for children with chronic pain syndromes; however, improved efficacy, compared with standard oral alternatives, has not been established. Due to the different kinetics of fentanyl in children, it may take longer to achieve the steady state in the skin depot, and the patch may not last for a full 72 hours. The patch must be removed if the patient undergoes magnetic resonance imaging (MRI) since patches contain metal and may cause burns to the skin. Withdrawal from the fentanyl patch may be delayed owing to availability of skin depot reserves. Unfortunately, an increasing number of patients are now being admitted to the PICU owing to fentanyl overdose as a result of chewing, smoking, or extracting fentanyl from the patch. A 12.5-μg fentanyl patch contains over 1 μg total of fentanyl.

Skeletal muscle rigidity after rapid administration of fentanyl is well described in the literature but can also occur with other synthetic opiates. It is mediated through the CNS and is an idiopathic response usually associated with a large bolus dose (≥5 μg/kg). It improves with the administration of NMBAs and is reversible with naloxone. Fentanyl has limited cardiovascular effects; moderate bradycardia is the most common. Fentanyl does not cause histamine release. Dosing in the ICU is either by bolus (1–2 μg/kg) or infusion (1–4 μg/kg per hour with higher doses required as tolerance develops). The short duration of effect of a single dose of fentanyl is not due to metabolism but rather to rapid redistribution. Maximum brain concentration after a bolus is achieved within 90 seconds. Then, because of rapid redistribution, the plasma level falls by 50% in 30 minutes. The result is a clinical duration of effect of a single dose of approximately 30 minutes. Fentanyl then accumulates in fat, where it is stored and slowly released with a longer elimination half-life of about 4 hours (longer than morphine). Marked respiratory depression occurs within 120 seconds, and a single dose of 5 μg/kg will cause apnea in 50% of patients. Fentanyl is metabolized by the liver to norfentanyl and hydroxyfentanyl derivatives, both of which are thought to be inactive. In the operating room, high-dose fentanyl is commonly used for cardiac anesthesia and for anesthetization of other unstable patients. A loading dose of 50 μg/kg, followed by 0.5 μg/kg per minute, will occupy all opioid receptors and produce a state of anesthesia. Many cases of awareness with patients under anesthesia have been documented, however, even when these high doses of fentanyl are used.
Sufentanil
Sufentanil is another synthetic opiate with actions and therapeutic effects similar to those of fentanyl. It is 5 to 10 times more potent than fentanyl and is the most potent opioid in clinical practice, posing a high risk of apnea with bolus administration. Dosing recommendations include a bolus of 0.2 to 0.4 μg/kg or an infusion of 0.2 to 1.0 μg/kg per hour.
After a single bolus, sufentanil has kinetics similar to that of fentanyl, with a short duration of clinical effect of approximately 30 minutes. However, with prolonged use, sufentanil accumulates less and is associated with a more rapid recovery after infusion because of its smaller volume of distribution and similar clearance. When the patient is receiving high doses of fentanyl, sufentanil can be useful to conserve infusion volume.
Alfentanil
Alfentanil is another synthetic opiate with a rapid onset. It is five times less potent than fentanyl. Although it is less lipid soluble than fentanyl because of its low p K a (negative logarithm of the acid ionization constant), a higher percentage of the drug is present in the active un-ionized form, which results in a rapid onset. Because of its low volume of distribution, alfentanil has a short elimination half-life, which results in a short duration of action (5–10 minutes). Dosing regimens include boluses of 5 to 10 μg/kg if there is spontaneous respiration or 20 to 50 μg/kg if the patient is mechanically ventilated. Alfentanil is a useful agent for preventing hypertension or increased intracranial pressure (ICP) responses to intubation. As with all synthetic opiates, there is a risk of muscle rigidity with higher dosing. Infusion dosing is typically 0.2 to 1.0 μg/kg per minute for patients receiving mechanical ventilation. Postinfusion recovery is quicker with alfentanil than with fentanyl. Alfentanyl is safe to use in patients with hepatic or renal failure.
Remifentanil
Remifentanil is one of the newest synthetic opiates in clinical practice. It was designed to be metabolized by plasma esterases for a short half-life. It is a potent μ-agonist with mild κ and δ effects. It is substantially more potent than fentanyl. Remifentanil is supplied as a white lyophilized powder that contains glycine and should not be used for epidural or spinal analgesia. The metabolism is by nonspecific esterases not affected by pseudocholinesterase deficiency. The metabolite, a weak μ-agonist, is excreted by the kidney. The kinetics of remifentanil is different from those of most opiates used in the ICU. It has a short half-life that is owing to metabolism rather than to redistribution. Therefore, remifentanil has what is known as a context-sensitive half-life. The elimination half-life for remifentanil is about 8 minutes. With an infusion of remifentanil, the half-life does not increase but rather remains constant. With opiates such as fentanyl and alfentanil, the clinical effect half-life increases with time until it reflects the elimination half-life of between 2 and 4 hours ( Fig. 132.3 ).

The kinetics reported for neonates is similar to those reported for adults. The continuous infusion rate depends on the degree of sedation/analgesia required (0.1–0.5 μg/kg per minute for sedation; 0.75–2.00 μg/kg per minute for balanced anesthesia; 4 μg/kg per minute for loss of consciousness). Remifentanil has effects on the cardiovascular system that are similar to those of fentanyl. Remifentanil causes a mild bradycardia and a slight decrease in blood pressure, which may be prevented with glycopyrrolate. No histamine release occurs. Remifentanil is a potent respiratory depressant. For spontaneous respiration, a low continuous infusion dose (without a bolus) should be used (0.1 μg/kg per minute). Sedation can be effectively managed by continuous infusion without the need for a bolus because of the short half-life. An increase or decrease of infusion rate is rapidly reflected by a change in the degree of sedation, which is important to note. Most other opiate sedatives require bolus dosing to achieve a rapid change in effect. This type of dosing is neither appropriate nor needed for remifentanil.
Remifentanil has the usual opiate adverse effects; however, because of the short half-life, these clinical effects are brief. Remifentanil may prove to be a safe and effective choice for PICU sedation in patients with severe renal or hepatic disease. However, the potential exists for glycine accumulation in patients with renal failure. It is an option for those who require overnight ventilation or for patients in whom a rapid awakening may be required for neurologic assessment. Remifentanil has been shown to reduce cerebral oxygen use and reduce cerebral blood flow (CBF) if the CO 2 is maintained in a normal range. Remifentanil is currently an expensive option and should not be considered for every patient. Because of its short duration, the postoperative patient may need an alternative analgesic after extubation. Rapid development of opiate tolerance with remifentanil has been described in healthy volunteers and in the ICU setting. This rapid tolerance has also been described in postoperative scoliosis patients. However, the increased morphine requirements described probably reflect the initial postoperative need to achieve an adequate morphine blood level rather than any acute tolerance.
Codeine
Codeine has a chemical structure similar to that of morphine. It has been used as an oral medication for cough suppression or mild to moderate pain relief. However, codeine itself has no analgesic effect; it is a prodrug that must be metabolized to morphine to promote analgesia. Between 10% to 20% of patients lack a metabolic pathway to convert codeine to morphine, which results in unpredictable effects and inadequate analgesia. Dosing is 0.5 to 1.0 mg/kg. Constipation is a major adverse effect, and some patients report having a vague, peculiar, or unpleasant feeling when they take codeine. Codeine can be habit forming. It can be given orally, IM, or rectally. Rapid IV use may result in cardiovascular collapse. Rectally administered codeine has been shown to have as rapid an onset as IM codeine, but it yields lower peak levels in children. Codeine has been the analgesic of choice by neurosurgeons because of the belief that pupillary signs are maintained with use of this drug. Morphine has been shown to be a more effective analgesic in patients with head injuries, however.
Approximately 1% to 2% of children have a duplication of the CYP2D6 allele that results in an ultrarapid-metabolizer phenotype. In these patients, a therapeutic dose of codeine that is rapidly converted to morphine can result in respiratory depression and death, especially following postoperative tonsillectomy in patients with a history of obstructed sleep apnea. This complication appears to be very rare in adults. Codeine now carries a black box warning, and its use in children is discouraged.
Hydromorphone
Hydromorphone is a hydrogenated ketone of morphine. It is seven times as potent as morphine, with a similar onset and duration of action. It causes less histamine release than morphine and may be used in patients who report pruritus due to morphine. Like morphine, hydromorphone undergoes hepatic metabolism. However, no active metabolites are dependent on renal excretion; thus, it may be an effective alternative in patients with renal insufficiency or failure.
Tramadol
Tramadol is an opiate analgesic. Its action is due to the metabolite O-desmethyltramadol, which relieves pain by binding to opiate receptors and by inhibiting the reuptake in the CNS and spinal cord of norepinephrine and serotonin, two pain-modifying neurotransmitters. Tramadol does not have antiinflammatory effects. Its use is indicated in cases of moderate to severe pain. Even though it is a narcotic-like agent, the FDA only recently classified tramadol as a controlled substance. Tramadol is now classified as a schedule IV medication, reflecting concerns about tolerance, habituation, abuse potential, and withdrawal syndrome. Dosage (not FDA approved for patients <16 years) is an initial oral administration of 1 to 2 mg/kg every 6 hours and should not exceed 6 mg/kg per day. An IV preparation is available outside of the United States. Patients with a creatinine clearance less than 30 mL/min should not receive more than one dose every 12 hours, with a maximum dose of 3 mg/kg per day. The dose for patients with cirrhosis or hepatic dysfunction is 1 mg/kg every 12 hours. Patients undergoing dialysis can receive their dose on the day of dialysis because only 7% of the drug is removed by the process. The adverse effects of tramadol most often involve the CNS and GI tract. Patients may become dependent on tramadol. Abuse is possible; thus, it should not be dispensed to opiate-dependent patients. Seizures have been seen in patients receiving high single oral doses of 10 mg/kg. This danger is even greater in patients with epilepsy and in anyone taking monoamine oxidase inhibitors or neuroleptic agents that lower the seizure threshold. Respiratory depression may occur if the recommended dosage is consistently exceeded or if another centrally acting depressant drug (e.g., alcohol) or an anesthetic is given concurrently. Because of the possibility of withdrawal symptoms, patients should not abruptly discontinue use of tramadol. Tramadol is not a useful drug for sedative action. Similar to codeine, it is metabolized by the CYP2D6 enzyme complex. Patients who are ultra-rapid metabolizers have an increased risk of respiratory depression and death due to high levels of the active metabolite O-desmethyltramadol. A black box warning akin to that for codeine is now in effect for tramadol. Current recommendations include the use of acetaminophen alternating with ibuprofen for initial analgesia treatment; oxycodone and hydrocodone can be considered when opiates are required.
Pharmacokinetics for relevant opiates are shown in Table 132.3 . Table 132.4 provides conversion doses for some commonly used oral opiates. A summary of IV doses of various opiates is provided in Table 132.5 .
Drug | Elimination Half-Life (h) | Volume Distribution (SS; L/kg) | Clearance (mL/kg/min) | Protein Binding (%) |
---|---|---|---|---|
Morphine | 2.2 | 3.3 | 15 | 30 |
Meperidine | 3.20 | 2.8 | 5.0 | 58 |
Fentanyl | 3.10 | 3.2 | 10.0 | 79 |
Sufentanil | 2.70 | 2.5 | 13.0 | 92 |
Alfentanil | 1.20 | 0.3 | 2.8 | 89 |
Remifentanil | 0.08 | 0.2 | 30.0 | 80 |
Drug | Oral | Parenteral |
---|---|---|
Morphine | 0.5 mg/kg q4h | 0.15 mg/kg q3h |
Hydromorphone | 0.1 mg/kg q4h | 0.02 mg/kg q4h |
Codeine | 4.0 mg/kg q3h | |
Hydrocodone | 0.5 mg/kg q3h | |
Oxycodone | 0.5 mg/kg q3h | |
Meperidine | 5.0 μg/kg q2h | 1.50 mg/kg q2h |
Fentanyl | 1.50 μg/kg q2h |
Drug | Relative Potency | Bolus Dose | Initial Infusion Rate | Active Metabolites |
---|---|---|---|---|
Morphine | 1.0 | 0.1 mg/kg | 0.04 mg/kg/h | M6G |
Meperidine | 0.1 | 1.0 mg/kg | N/A | Normeperidine |
Fentanyl | 100.0 | 1.0 μg/kg | 1.0 μg/kg/h | None |
Hydromorphone | 7.0 | 0.015 mg/kg | 0.005 mg/kg/h | None |
Sufentanil | 500.0 | 0.2 μg/kg | 0.2 μg/kg/h | None |
Remifentanil | N/A | N/A | 0.1 μg/kg/min | None |
Alfentanil | 10.0 | 10 μg/kg | 10 μg/kg/h | None |
Methadone | 1.0 | 0.1 mg/kg | N/A | None |
Opiate antagonists
Several opiate antagonists are available. The most commonly used is naloxone, which is a specific and sensitive receptor antagonist of all opiate receptors. It is administered in either low doses (1 μg/kg) for partial opiate reversal or to ameliorate opiate-induced pruritus, or in higher doses for full opiate reversal (10 μg/kg) or emergency reversal of opiate overdose (100 μg/kg). If the drug cannot be administered intravenously, then it can be given intramuscularly, intranasally, or into the midventral surface of the tongue. When naloxone is being used to antagonize a long-acting agonist, an infusion may be necessary because its half-life is only 30 to 81 minutes (mean of 64 ± 12 minutes). In neonates, the half-life has been reported as 3.1 ± 0.5 hours. However, this prolonged effect is likely to be offset by a concomitant increase in the duration of action of the opioid for which the naloxone is given. No effect is seen in the healthy patient in the absence of administered opioids; however, in the setting of sepsis in the ICU, a vasopressor effect may occur, presumably because of an interaction with endogenous opioids released in response to stress. Nalmefene, a longer-acting antagonist, can be given through IV, IM, and SC routes. It has a redistribution half-life of 41 minutes and a terminal half-life of 10.8 hours in adults and somewhat less in children. Reappearance of the antagonized opioid is unlikely if nalmefene is given in an adequate dose.
There has been a growing interest in the use of opiate antagonists in the management of opiate-induced ileus. Naloxone can be given orally. However, there is evidence of a central effect that slightly reduces the efficacy of parenterally administered opiates. Two opiate antagonists are available that have been approved for opiate-induced constipation. Naloxegol is a PEGylated naloxone that does not cross the blood-brain barrier, inhibits opioid receptors in the GI tract, and improves motility. Methyl naltrexone is also approved for opiate-induced constipation. These drugs are not routinely used; however, they may be added to bowel regimens to prevent opiate-induced bowel dysfunction.
Incidental pain syndromes in the pediatric intensive care unit
Although the techniques used to sedate children are often applied in order to facilitate their PICU care, many children have pain that is related to their underlying condition. Many options are available for controlling pain in the pediatric patient ( Box 132.2 ). The pharmacologic management of pain should follow the traditional World Health Organization analgesic ladder, which begins with a nonopioid analgesic such as a nonsteroidal antiinflammatory drug or acetaminophen, followed by a weak opioid, such as hydrocodone added to the nonopioid, and then a strong opioid such as morphine or hydromorphone as needed. When taken orally, a sustained-release preparation is useful once the dose requirement has been determined. The required dose can be variable in the patient taking opioids for a prolonged period; not appreciating this variability is a common cause for therapeutic failure. In addition, once a dose requirement is known, the analgesic should be given to preempt pain rather than to relieve pain as required. At each level of analgesic use, the addition of adjuvant medications should be considered. Adjuvant drugs fall into six groups: antidepressant, anticonvulsant, neuroleptic, steroid, stimulant, and local anesthetic. Of the tricyclic antidepressants, nortriptyline is available in a liquid form. The tricyclic antidepressants are indicated for neuropathic pain, particularly when the patient describes a burning pain. Anticonvulsant agents, gabapentin, pregabalin, and carbamazepine can be useful for neuropathic pain as well. These drugs often work best when the pain is described as shooting or lancinating. Neuropathic pain may result from tumor invasion, vincristine therapy, cytomegalovirus infection, or human immunodeficiency infection. Neuroleptic drugs, including chlorpromazine and trimeprazine, may be useful in the management of nausea, anxiety, and pruritus. Steroids benefit mood, inflammation, nausea, appetite, nerve swelling/entrapment, and vasculitis. When opioid sedation is interfering with quality of life, a stimulant such as an amphetamine may restore energy and alertness while allowing ongoing analgesia from the opioid. Pain can sometimes be managed by local anesthetic, placed by peripheral nerve block, topically, or as a neuraxial block.
Analgesics
Opioids—weak, potent
Nonsteroidal antiinflammatory drugs
Nonopioids
Anesthetics
Regional block
Epidural anesthesia
Topical anesthesia
Physical
Thermal
Massage
Physical therapy
Transcutaneous electrical nerve stimulation
Cognitive
Imagery
Distraction
Hypnosis
Choices and control
Information
Role play
Behavioral
Biofeedback
Relaxation therapy
Patient-controlled analgesia (PCA) has become the mainstay of postoperative pain relief in children because of its efficacy and safety. However, its use is limited by the child’s ability to understand how to use the PCA pump. Proxy PCA (PCA-P) has been used for younger children or those with cognitive impairment. The use of PCA by the nurse or the caregiver may override the safety net that the PCA has. The use of PCA-P has been associated with greater need for rescue interventions; however, it is often used in sicker children. When PCA-P is used, careful evaluation and rigorous monitoring are needed.
Sickle cell crisis
Pain in patients with sickle cell disease differs from that experienced by patients with cancer or acquired immune deficiency syndrome in that intermittent episodes of severe pain occur, requiring urgent intensive treatment. Chronic pain is present because of long-term tissue and bone damage from periods of ischemia during past crises, including persistent myocardial ischemia. Patients may be receiving long-acting opioids or may have had repeated exposure to opioids with past crises. Chest crises result from the sickling of erythrocytes in the pulmonary microvasculature and result in hypoxia to the rest of the body and local lung damage. The systemic hypoxia worsens the crisis; thus, it is self-perpetuating. Chest radiograph changes may lag other symptoms, and an associated paralytic ileus may be present.
Poor pulmonary function may incorrectly discourage the practitioner from using adequate opioid analgesics out of concern for worsening the hypoxia. However, it is important not to underestimate the need for pain relief and to appreciate that past opioid exposure may have resulted in tolerance to opioids. It is important that the pain is assessed early and quickly treated. Pain must be reevaluated often to ensure that additional doses are given in a timely manner. When considering the use of PCA, appropriate dosing must be employed as well. Inadequately low dosing will result in unsatisfactory analgesia in patients who are not opiate naïve. These patients require frequent pain reevaluation to ensure that the PCA is programmed properly. If IV access is difficult to obtain, morphine may be given subcutaneously or orally. Ketamine has anecdotally been suggested as an adjunct in sickle cell pain crisis, with purported improvement in pain scores and a reduction in opiate requirements. Intranasal fentanyl has also been used with a reduction in pain within 20 minutes. However, the analgesic effect was not sustained for long and the use of intranasal opiates may complicate the dosing of subsequent parenteral opiates. Its use in the emergency department reduces the time to parenteral opiate when compared to IV administration, without any noted complications. Codeine is the only schedule 3 opiate in the United States, and its use is still common. In a study looking at CYP2D6 genotypes in a sickle cell population, approximately 7% of patients were possible or actual ultra-rapid metabolizers and 1.4% were poor metabolizers. While safe in typical subjects, codeine must be avoided in both of these subsets. Anecdotal success also has been reported with nebulized morphine.
Opiate tolerance
The use of opiate infusions in the ICU is associated with the potential for the development of tolerance or dependence. This can be due to receptor desensitization or upregulation of the postreceptor pathways, which mostly results in a physiologic dependency state. The need to wean sedatives to facilitate ventilation weaning and extubation further compounds this problem, resulting in iatrogenic withdrawal syndrome (IWS), which has been shown to delay patient recovery and prolong hospitalization. IWS can occur if an opiate is discontinued abruptly. Withdrawal effects have been correlated to the total dose and duration of fentanyl infusion. A fentanyl infusion of 5 days or a total cumulative dose of 1.6 mg/kg during the hospital stay was associated with a 50% chance of developing narcotic withdrawal, whereas a fentanyl infusion of 9 days or longer or a total cumulative fentanyl dose of 2.5 mg/kg or more during the hospital stay had a 100% incidence of withdrawal. The rising plasma fentanyl levels caused by increased dosing suggested that increased metabolism or clearance is not responsible for the development of tolerance. A study of patients in a PICU conducted to determine the degree of opiate tolerance showed a significant increase in opiate dosing required for adequate sedation. Opiate dosage increased by about 80% per week for the first 3 weeks of opiate use. No difference in the rate of opiate increase was found with respect to age, postoperative status, mode of ventilation, and use of NMBAs.
For patients considered to be at risk of withdrawal, several options are available. If circumstances allow, it is better to start the treatment for withdrawal prevention before the patient develops symptoms and signs of withdrawal. Opiate withdrawal is not usually a serious medical problem; although highly uncomfortable, it is rarely life-threatening and is self-limited. However, treatment should begin as early as possible for patient comfort. The hypersympathetic state associated with opiate withdrawal may be detrimental to the patient. The signs and symptoms of withdrawal are nonspecific; other causes—such as infection, hypoglycemia, hypocalcemia, hyperthyroidism, and hypoxia—should be excluded. Because of the nonspecific nature of the symptoms of opiate withdrawal, several scoring systems have been described to aid with the diagnostic process. The Finnegan score is based on 31 variables and is lengthy. The Lipsitz score is shorter and easier to use. Both of these scoring systems, however, were devised for use with neonates; several of the measurements are not appropriate for patients in the PICU. The Withdrawal Assessment Tool-1 (WAT-1) has been developed and validated in a PICU population. The score is quick and easy to calculate ( Table 132.6 ), usually done once a shift. IWS is considered when the WAT-1 score is 3 or greater. The risk of withdrawal with respect to this tool appears to be related to both opiate dosing and duration. The score has features typical of opiate withdrawal, such as sweating, yawning, and loose stools. The score also takes into account agitation, tremor, and uncoordinated movements, which are more often associated with BZD withdrawal. The signs related to sympathetic overactivity that may be associated with opiate withdrawal, such as tachycardia and hypertension, were not found to be specific enough to be included in this assessment tool. The tool appears to be accurate, with an area under the curve of 94% (sensitivity of 87% and specificity of 88%). ,
Symptom/Sign | Score Assigned a |
---|---|
Loose stools | 1 |
Vomiting or gagging | 1 |
Temperature >37.8°C | 1 |
Awake and distressed prior to stimulation | 1 |
Moderate or severe tremor | 1 |
Sweating | 1 |
Uncoordinated/repetitive movements | 1 |
Repeated yawning or sneezing | 1 |
Poststimulation (in order, if no response) | |
Call patient’s name. If no response, touch patient’s arm. If no response, perform a planned uncomfortable procedure. | |
Startle response | 1 |
Increased muscle tone | 1 |
How quickly does the child settle? | |
| 1 |
| 2 |
The other tool that has also been validated in the PICU for withdrawal assessment is the Sophia Observational Score (SOS). It is quite similar to the WAT-1, as it uses 15 items and includes both abnormal heart rate and respiratory rate. Additional components include inconsolable crying, hallucinations, and sleep disorder. The accuracy of the score appears to be very similar to the WAT-1, with an area under the curve of 95% (sensitivity of 83%, specificity of 93%). Both scores have good intra- and interrater reliability and should be useful in the management of opiate withdrawal as well as BZD withdrawal in the PICU.
Several therapeutic options are available for the prevention and treatment of opiate withdrawal. Drugs from the same class are preferable. The FDA has approved methadone for the treatment of opiate withdrawal. Other agents that may be useful include morphine, clonidine, dexmedetomidine, phenobarbital, paregoric, chlorpromazine, the transdermal clonidine patch, and SC fentanyl. Paregoric contains morphine plus papaverine, noscapine, camphor (a CNS stimulant), ethanol (45%), benzoic acid (which competes with bilirubin-binding sites), and glycerin (which causes diarrhea). Paregoric has been used for neonatal withdrawal, but because of its composition, it may cause adverse effects. Chlorpromazine may be useful for GI adverse effects, but hypothermia and hypotension may occur. Haloperidol may be of use, having minimal respiratory depression and no active metabolites. It also offers cardiovascular stability. Phenobarbital has been used for hyperactive behavior; however, it can cause significant CNS depression, induces drug metabolism, and is tolerance/dependence forming.
Methadone is the most suitable agent for treating opiate withdrawal. It has an oral bioavailability of 80% to 90% and an elimination half-life of 12 to 24 hours. It is equipotent to morphine. Methadone has inactive metabolites and is less sedating than morphine while remaining an effective analgesic. Because of its higher bioavailability and reduced first-pass metabolism, the effect of oral doses is more predictable than that of morphine. Methadone has been extensively used in the outpatient management of opiate addiction.
The convenience of the oral route, the less-frequent dosing because of its longer half-life, and the ease of calculating doses because of its equal potency to morphine make methadone attractive for use in the management of opiate withdrawal in children. However, significant variability exists in recommendations regarding the methadone dose that should be used to prevent opiate withdrawal in children. Several factors are important in the dosing of methadone for withdrawal from fentanyl. After prolonged IV administration, fentanyl has a potency 100 times that of methadone; it has a metabolic half-life approximately one-quarter that of methadone; and if given intravenously, it has a bioavailability 20% greater than orally administered methadone. A study assessing the effectiveness of a fentanyl-methadone conversion protocol found that administering 2.4 times the daily fentanyl dose as methadone successfully prevented withdrawal symptoms. In that study, methadone was given intravenously for 24 hours; the fentanyl dose was decreased by 50% on day 1 and by another 50% on day 2, then discontinued. On day 3, methadone was converted to oral dosing. Methadone was given intravenously initially (with the possibility of administering additional doses, as needed) because it could take up to 5 days to reach a steady state with scheduled oral dosing due to its long half-life. The duration of methadone treatment varied from 1 to 4 weeks, depending on the duration of the preceding opiate infusion. Methadone was weaned by 3% to 15% per day with no signs of withdrawal. To date, there have been no published cases of respiratory arrest when methadone has been used for opiate weaning.
The incidence, risk factors, and best management strategy for opiate withdrawal in the PICU are still open to debate. Several articles have suggested risk factors for withdrawal. Younger age, higher level of critical illness, duration, and cumulative dose are important patient variables, but not all studies demonstrate these same risk factors. Postoperative patients appear to be less likely to develop tolerance, as do postoperative patients who received morphine rather than fentanyl. However, in nonsurgical patients, the opiate chosen was not significant. Of patients receiving opiates for more than 4 days, 35% required the dose to be doubled by 14 days. Most of these increased dose requirements occurred within 3 to 6 days. Starting at a lower dose was also a risk factor for increased dose requirements. In this study, however, the opiate doses used were very low, with a peak fentanyl dose of only 4 μg/kg per hour. Thus, these factors may not apply in patients receiving higher doses. Of interest, the concomitant use of midazolam infusions also was associated with increased opiate needs; whether this reflects a patient factor or a drug effect is unknown. Caution should be taken when interpreting these data given the multicenter nature of the study with the lack of a sedation protocol in these institutions. There is some belief, but little evidence, that using sedation protocols can reduce this problem. Intermittent rather than continuous infusions can reduce the risk. However, this option has to be balanced against the primary aim of sedation, which is patient comfort and safety.
Finally, hospital-based factors can play a part. It would appear prudent to initiate the conversion from fentanyl/morphine to methadone in the ICU environment in the event that problems arise and to ensure that an adequate dose is given. Once stabilized, the patient may be transferred out of the ICU and ultimately home, with a clear plan for decreasing the methadone dose over time. The weaning plan should involve the primary pediatrician as an additional safeguard.
An alternative to fentanyl equivalence-based methadone dosing has also been reported. In this study, the patients received either a fixed low dose (0.1 mg/kg) of methadone or a high dose (fentanyl dose [μg/kg per hour] × 0.1 × weight) of methadone. The methadone was tapered in a fixed manner irrespective of the duration of fentanyl infusion. There was no difference in the success (about 60%) of the methadone taper between groups. However, four times as many patients in the high-dose methadone group became oversedated. The success rate of either of these protocols appears to be poor, possibly due to the fairly rapid wean of the methadone irrespective of the duration of opiate exposure. Of note, the peak fentanyl dosing was low (4 μg/kg per hour) and the cumulative dose was also low (0.59 mg/kg), both below what are considered high risk for IWS. This is an important consideration because, if using the high-dose strategy, the methadone dose based on a much higher fentanyl rate would expose the patient to very high doses of methadone and risk respiratory depression. Also, methadone accumulates over several days—this respiratory depression could occur when the child is not being monitored as closely, after transfer to the floor. This study supports the use of the lowest dose possible to prevent withdrawal, which is safer and allows for a shorter methadone taper period. If the dose is found to be insufficient, small (25%) adjunct doses of methadone can be given to control the withdrawal symptoms. Then, if needed, the methadone dose can be modified to reflect the patient’s actual requirements. In younger children, an every 8- or 12-hour dosing schedule may not be adequate; a change to an every 6-hour regimen may be required in the initial few days. Again, caution must be observed with respect to drug accumulation during this period. The purpose of methadone is to prevent withdrawal symptoms and keep the child comfortable. The methadone dose to prevent withdrawal is usually lower than the sedation dose. Patient safety is paramount—opiate withdrawal, although unpleasant, is not life-threatening and, with appropriate assessment and timely intervention, can be corrected quickly. Excessive sedation should be avoided.
Lofexidine has been used as an oral adjunct during outpatient opiate withdrawal and is approved by the FDA for that purpose. α 2 -Agonists are appropriate for opiate withdrawal in the ICU setting. There have been several reports of either SC or IV dexmedetomidine infusions (0.5 μg/kg per hour) that have demonstrated the ability to reduce the symptoms of opiate withdrawal in PICU patients. If already on dexmedetomidine, extubating on a lower infusion rate may result in a lower dose of methadone required to alleviate withdrawal symptoms.
The use of a clonidine patch also has been evaluated in the PICU. Clonidine has been shown to be effective in the management of nicotine, opiate, and alcohol withdrawal. It decreases sympathetic outflow from the CNS and has a synergistic effect for analgesia, both at the central and spinal levels. In one report, a sustained-release transdermal clonidine hydrochloride patch (mean, 5.8 μg/kg per day; range, 4.2–8.5 μg/kg per day) was applied to 8 consecutive patients after tracheal reconstructive operations and 7 days of mechanical ventilation before discontinuation of sedative infusions and scheduled extubation. One patch had to be removed because of hypotension. The transdermal clonidine patch seemed to be safe and efficacious in preventing withdrawal. Its use is attractive because of its noninvasive approach. However, the use of a transdermal patch prevents titration of the effect. In addition, problems with bradycardia, hypotension, hypothermia, sedation, and dysrhythmia may occur.
A confounding issue in many publications and in the clinical management of opiate withdrawal is the potential for simultaneous BZD withdrawal. Most researchers have not been able to successfully separate these two issues. The symptoms of BZD withdrawal differ from those of opiate withdrawal because the BZD symptoms generally include less sympathetic activation. BZD withdrawal symptoms are characterized by agitation and a movement disorder. If BZD withdrawal is a concern, low-dose lorazepam or diazepam should be added to the withdrawal management strategy ( Table 132.7 ). A prospective study of BZD withdrawal following lorazepam infusion (up to 0.3 mg/kg per hour) documented BZD withdrawal syndrome in approximately 25% of the children. This withdrawal occurred even when using a 6-day tapering of the lorazepam dose. All of the children had been previously weaned off fentanyl infusions. No predisposing risk factors were found for BZD withdrawal with respect to BZD or opiate dosing or duration.
Drug | Elimination Half-Life (h) | Volume Distribution (SS; L/kg) | Clearance (mL/kg/min) | Protein Binding (%) |
---|---|---|---|---|
Diazepam | 46.60 | 1.13 | 0.4 | 97.8 |
Midazolam | 3.00 | 1.09 | 7.5 | 94.0 |
Lorazepam | 14.50 | 1.10 | 1.1 | 91.0 |
Flumazenil | 0.67 | 1.20 | 15.3 | 50.0 |
Rapid opiate detoxification
There are various strategies for rapid opiate detoxification in the ICU. These have employed a form of deep sedation (with propofol or another anesthetic agent) to facilitate opioid withdrawal in patients addicted to the recreational use of opiates. The patients are given high doses of opiate antagonists to displace all opiates from their receptors while under heavy sedation to reduce the occurrence and effects of the sympathetic stimulation observed with short-term opiate withdrawal. These procedures have been safely performed in the ICU. However, major complications can occur when these procedures were not performed with full ICU support. The effectiveness and safety of 1-day opiate detoxification are still a matter of debate. If used, it should be combined with a comprehensive support plan to optimize long-term success.
In the PICU, deep sedation with propofol has been used to facilitate rapid opiate weaning of ventilator-dependent patients. The use of propofol for up to 3 days allowed a reduction of fentanyl dosing from 24 to 9 μg/kg per hour (a 65% reduction), with no signs or symptoms of opiate withdrawal or metabolic acidosis. Opiate antagonists were not used for this rapid weaning process. However, concern has been raised regarding the long-term administration of propofol, especially in the PICU patient, given the potential for propofol infusion syndrome.
Benzodiazepines
BZDs are among the most commonly used agents for sedation in the ICU. They augment the function of the GABA type A (GABA A ) receptor at the postsynaptic membrane. This pentameric protein controls a chloride channel, the opening of which leads to an inhibitory effect due to hyperpolarization of the cell membrane. , BZDs bind to BZD receptors, which are usually found as part of the GABA A receptor in the CNS, enhancing the effect of endogenous GABA. Peripheral BZD receptors are not usually associated with the GABA A receptor but are a binding site for diazepam and midazolam. These 18-kDa proteins are associated with regulation of cellular proliferation, immunomodulation, porphyrin transport, heme biosynthesis, and anion transport. In particular, they seem to be important in the regulation of steroid synthesis and apoptosis, and they have a significant effect on the hypothalamic-pituitary-adrenal axis. These latter effects may be pertinent to the physiologic care of patients in the ICU.
BZD receptors are bound by a family of endogenous peptides called endozepines, which have similar effects to the BZDs. , The expression of this diazepam-binding inhibitor may be relevant to the development of dependence not only on BZDs but also on alcohol and opioids. Therefore, it may be relevant in the drug dependence commonly seen in patients in the PICU who are given these agents continuously. Naturally occurring BZDs have been detected with structures similar to those used clinically. Subsets of GABA A receptors have been shown to have different effects. Type 1 receptors are responsible for sedation and anterograde amnesia, whereas type 2 receptors mediate anxiolysis. It may be possible to develop selective subtype receptor agonists to provide anxiolysis without sedation, amnesia, or dependence.
The general pharmacologic effects of BZDs are sedation, anxiolysis, euphoria (limbic system), anticonvulsive, reduced skeletal muscle tone (through spinal BZD receptors), and neuroendocrine effects. They impair acquisition and encoding of new information, providing antegrade amnesia. They do not have any analgesic properties and little direct effect on ICP. Their effects are dose dependent. Patient cofactors—including age, concurrent disease, and any co-sedation therapy—influence responses to BZDs. Paradoxical reactions are reported, in which agitation rather than calm is observed. In healthy patients, BZDs have few cardiovascular adverse effects. However, profound cardiovascular depression may be observed in a critically ill patient. Thus, BZDs should be used judiciously until the patient response is known. Midazolam has been most often associated with this effect, and research in dogs has shown both negative inotropy and chronotropy, especially when the sympathetic response has been abolished.
Specific benzodiazepines
Diazepam
The first widely used BZD in the ICU was diazepam. Because of its low solubility in water, it is available in the IV or IM form dissolved in propylene glycol. This formulation may cause pain and thrombophlebitis with peripheral IV use. A lipid emulsion that has fewer adverse effects is available in the United Kingdom. Diazepam is inexpensive and effective for short-term sedation; in such cases, accumulation is less of a concern. Diazepam may be given orally because it has good absorption, but absorption is less predictable when given rectally or intramuscularly. It is highly lipid soluble, with a long half-life (24 hours). Metabolism by oxidative biotransformation generates several hypnotically active metabolites with a long elimination half-life, including oxazepam (half-life, 10 hours) and N-dimethyldiazepam (half-life, 93 hours). Delayed recovery has been reported in neonates after receiving diazepam, possibly because of the long half-life of dimethyldiazepam. Prolongation of effects occurs in patients when clearance is reduced because of hepatic dysfunction and when metabolism is inhibited by drugs such as cimetidine and omeprazole.
BZDs are used as first-line therapy for pediatric convulsive seizures or status epilepticus. Diazepam has been safely used rectally or intravenously to prevent and treat seizures. , Recent evidence supports that other BZDs (midazolam and lorazepam) are more effective than diazepam for treating convulsive seizure or status epilepticus. Diazepam is used in the treatment for spasticity in children with cerebral palsy and other illnesses. In a recent randomized prospective follow-up study, Goyal et al. compared outcome of oral diazepam and baclofen in children with spastic cerebral palsy. The mean Modified Ashworth Scale (MAS) score improved from 1.96 ± 0.4 at baseline to 1.63 ± 0.40 and 1.41 ± 0.36 at 1 month and 3 months for diazepam and from 1.84 ± 0.64 to 1.57 ± 0.59 and 1.31 ± 0.48, respectively for baclofen ( P = .0001). There was a significant improvement in the range of motion in both groups. Both diazepam and baclofen were safe to use.
Midazolam
Midazolam is an imidazobenzodiazepine. It has a short elimination half-life of 2 hours and is water soluble, which means that IV injection is nonirritating. Because of these factors, it has become popular in ICUs for sedation by infusion. Intranasally (0.2 mg/kg), midazolam has proved to be as effective at controlling febrile seizures as IV diazepam (0.3 mg/kg). Intramuscular midazolam can be as safe and effective as IV lorazepam in managing patients with status epilepticus. Midazolam has extensive first-pass metabolism and provides less reliable results when given PO. Despite that, this route is often successfully used for premedication of children before general anesthesia in doses of 0.5 to 0.75 mg/kg (maximum, 20 mg). It is available in a pleasant-tasting cherry syrup and is effective in 10 to 15 minutes, providing up to 1 hour of adequate anxiolysis, although residual hangover effects may persist. Rectal and sublingual administration have also been described.
Midazolam is considered one of the mainstays of continuous infusion therapies in the management for refractory status epilepticus in pediatric patients. A bolus dose of midazolam ranging from 0.1 to 0.5 mg/kg is used at the start of treatment followed by a continuous infusion rate of 0.06 to 0.5 mg/kg per hour (up to 1.5 mg/kg per hour) or 1.0 to 8.3 μg/kg per minute (up to 25 μg/kg per minute). Higher infusion rates of midazolam are used to treat EEG seizure activity in comparison to clinical convulsive seizure activity. Significantly higher dosing rates are used when burst suppression is the chosen end point.
IV and nasal midazolam have been successfully and safely used for imaging procedural sedation. The success rate is higher for shorter imaging procedures, such as for computed tomography scan versus MRI. Midazolam is about eight times more potent than diazepam, with sedation starting dose recommendations of a bolus dose of 0.05 to 0.1 mg/kg and an infusion of 1 to 6 µg/kg per minute.
Midazolam is metabolized by the cytochrome P450 system subfamily IIIA (nifedipine oxidase), polypeptide 4 (CYP3A4), to hydroxymidazolam (63% potency) and hydroxymidazolam glucuronide (9% potency). Because of the high degree of protein binding (94% protein bound), the free level can be significantly changed with interactions because of the protein binding, which also may occur with heparin. Hepatic or renal failure increases the free fraction by two to three times, and its effect also can be prolonged by the accumulation of active metabolites. The half-life of midazolam in patients in the ICU may be prolonged compared with that in healthy patients. With short-term infusions (<12 hours), it retains a rapid recovery; however, with increased duration of use, recovery becomes prolonged. Its clearance may be reduced by several commonly used ICU drugs, including calcium channel blockers, erythromycin, and triazole antifungal agents.
Midazolam clearance can also be reduced by mechanical ventilation, possibly due to reduced cardiac output. There appears to be no correlation between day-night cycle and midazolam pharmacokinetics in critically ill children. Critical illness itself can have a major effect on midazolam clearance. Using pharmacokinetics modeling, midazolam clearance was found to be lower in critically ill patients when compared with healthy infants admitted for postoperative monitoring. This is believed to be owing to reduced cytochrome P450 3A4/5 (CYP3A4/5) activity as a result of inflammation, reduction in liver blood flow as a result of mechanical ventilation or acute illness, and the reduction of total albumin that is frequently reported in critically ill patients.
Lorazepam
Lorazepam is an alternative water-soluble agent that is well absorbed after both oral and IM administration. It produces sedation for 4 to 8 hours after a single dose. Lorazepam has a slower onset than does midazolam. The elimination half-life is about 14 hours. Metabolism is by glucuronyl transferase, not cytochrome P450, and there are no active metabolites. This metabolism is unaffected by cimetidine or phenobarbital, which affects only oxidative metabolic pathways. Sodium valproate may inhibit its metabolism. In patients with advanced liver disease, these phase II glucuronidation reactions are better preserved, and the increased half-life seen is due to increases in the volume of distribution rather than to reduced clearance. In patients with renal failure, prolonged half-life is also due to reduced protein binding because clearance is unchanged. No change in metabolism occurs with aging or critical illness. In a comparison of infusions of midazolam and lorazepam, recovery characteristics were found to be significantly different. In patients receiving lorazepam, it took an average of 260 minutes to return to baseline; in patients receiving midazolam, it took more than six times longer to return to baseline.
Lorazepam may be administered by bolus (0.05–0.10 mg/kg every 2 to 4 hours) or by infusion (0.05 mg/kg per hour). Lorazepam is slightly less expensive than midazolam. It has been recommended as the BZD of choice for long-term sedation because of its more predictable recovery profile in patients in the ICU. Lorazepam for IV use has propylene glycol as a diluent; thus, there is risk of metabolic lactic acidosis with prolonged use or high daily doses. Several other potential ICU drugs may use propylene glycol as a carrier, including some IV preparations of phenytoin and phenobarbital, nitroglycerin, digoxin, and etomidate. Propylene glycol toxicity has been reported in adults who received infusions of multiple medications containing propylene glycol. Thus, care should be taken when lorazepam is infused in conjunction with those drugs. In critically ill children, propylene glycol levels correlate well with the dose of lorazepam administered; however, no metabolic abnormalities were detected. Hemodialysis has been used successfully in the management of the lorazepam-associated propylene glycol toxicity.
All of these BZDs have been commonly used as the first-line treatment for pediatric patients with status epilepticus. The use of the longer-acting lorazepam may preclude the need for additional dosing for recurrent seizures. However, there may be an increased risk of respiratory depression, especially if redosing is required. Some earlier studies have indicated the safety of lorazepam over diazepam, and one study suggested that the use of IV lorazepam (0.1 mg/kg) is as safe and efficacious as IV diazepam (0.2 mg/kg) in pediatric patients with status epilepticus.
IM lorazepam can be an ideal choice in children with complex medical and physical disabilities when IV access is a challenge. A recent retrospective descriptive study reported resolution of status epilepticus in 68.5% of episodes after one dose of IM lorazepam 0.05 to 0.1 mg/kg in this patient population in a single institution. The majority of children at that facility did not have IV access, requiring the use of alternative routes of administration of BZDs for acute seizure management. The pharmacokinetics for different BZDs is shown in Table 132.7 .
Tolerance for and dependence on benzodiazepines
Tolerance for and dependence on BZDs can occur as with opiates in the PICU. This effect is not all due to receptor number downregulation. The incidence of IWS secondary to BZDs ranges from 17% to 35% in pediatric critical care units. Risk of IWS increases once continuous BZD/opioid infusion time lasts longer than 5 days and warrants close monitoring for signs and symptoms. Monitoring for IWS should start as early as after 3 days of continuous infusion; a midazolam infusion rate greater than 0.35 mg/kg per hour is predictive of IWS.
Withdrawal symptoms may be avoided with a slow taper of the medication of 10% per day or by substituting with a long-acting oral agent, such as diazepam or lorazepam. Acute BZD withdrawal symptoms may include anxiety, insomnia, nightmares, seizures, psychosis, and hyperpyrexia. A postmidazolam infusion phenomenon has been described that includes poor social interaction, decreased eye contact, and a decreased interest in the surroundings. The patient may exhibit choreoathetotic movements with dystonic posturing that can persist for 2 to 4 weeks but resolves without sequelae. As would have been anticipated, the longer the duration and higher the cumulative dose of BZDs, the higher the chance that withdrawal symptoms will occur.
Flumazenil
Flumazenil is an imidazobenzodiazepine and is a specific competitive antagonist of the BZD receptor. It has no effect on other drugs such as barbiturates, ethanol, or other GABA-mimetic agents. Flumazenil reverses the hypnotic and sedative effects of BZDs. It is primarily used to reduce the residual effects of BZD, including sedation, amnesia, respiratory depression, and muscle relaxation. Flumazenil was also reported to act as an antagonist of sevoflurane, isoflurane, and propofol to shorten anesthesia emergence. This effect was also considered to be related to competitive binding to the GABA receptor. , It has a half-life of approximately 1 hour after a single IV bolus. In patients with hepatic impairment, its half-life and clearance are prolonged, and a significant increase (>50%) of free drug occurs because of reduced plasma protein binding. Renal failure has little effect on the pharmacokinetics of flumazenil. It is indicated for the complete or partial reversal of the central sedative effects of BZDs. Contraindications include patients who have a known hypersensitivity to BZDs, patients with epilepsy who are receiving treatment with BZDs, and patients who have overdosed with a tricyclic antidepressant. Flumazenil use often is associated with mild to moderate tachycardia and hypertension.
In cases of multiple-drug overdose, the use of flumazenil remains controversial. It often is overused in the emergency setting without due concern for potential adverse reactions because of the potential toxic effects (e.g., cardiac arrhythmias or convulsions) of other psychotropic drugs ingested. The toxicity of tricyclic antidepressants may become apparent as the effects of BZDs are antagonized. Patients should be evaluated for the signs and symptoms of a tricyclic antidepressant overdose. An electrocardiogram (ECG) may be helpful in determining the risks involved.
Flumazenil dosing information for pediatric patients is limited. The initial suggested dose is 0.01 mg/kg (maximum, 0.2 mg) with incremental doses of 0.005 to 0.010 mg/kg (maximum, 0.2 mg) given every minute up to a maximum cumulative dose of 1 mg. The lower doses are suggested for sedation reversal and the higher doses for BZD overdose. Infusions at 0.05 to 0.1 mg/kg per hour have been used. The use of flumazenil in sedated patients in the ICU should be tempered by the potential for an unrecognized BZD dependence, which would increase the risk of adverse effects. If its use is required, a carefully titrated dose would be appropriate. The half-life of flumazenil is much shorter than that of some of the BZDs it may be counteracting (see Table 132.7 ). The use of an infusion may be necessary because re-sedation has been reported after single-bolus use. However, this requirement should not preclude the use of flumazenil in an ICU setting. Flumazenil has been used for the reversal of moderate sedation. The onset is usually within 1 to 2 minutes, with an expected peak effect at 6 to 10 minutes after IV injection. Both the plasma concentration of BZDs and the flumazenil IV dose determine the duration of drug reversal. Although well tolerated in the pediatric population, flumazenil was not shown to significantly reduce recovery time. Because flumazenil has a limited duration with the potential for re-sedation after discharge from medical care, an appropriate period of observation is required. A study in which researchers monitored the effects of flumazenil after sedation indicated that some of the residual effects of midazolam were still present after reversal. Flumazenil has been used to treat a paradoxical midazolam reaction , and shown to be effective in the management of hepatic encephalopathy or hyperammonemia. In addition to the IV route, flumazenil has been administered by rectal, nasal, IM, and oral routes. Higher doses are required for rectal (50 μg/kg) and intranasal (40 μg/kg) routes. Oral flumazenil is not a good option because of slow onset and poor bioavailability (16%) owing to the first-pass effect in the liver.
Other agents for sedation in the pediatric intensive care unit
Chloral hydrate
Chloral hydrate is a widely used oral hypnotic/sedative agent. It has been used for sedation in radiographic procedures, EEGs, and many healthcare locations. It was first synthesized in 1832 and used in 1869 as a hypnotic agent. Shortly after, reports of acute and chronic toxicity were published. In 1910, it was labeled as the most dangerous of hypnotics, even though heroin and opium were in common use at that time. The addition of ethanol potentiates its effect (street name “Mickey Finn”). It has been used to control agitation in the intensive care nursery and to treat sleep difficulties in older patients.
Chloral hydrate is rapidly and completely absorbed from the GI tract and is immediately converted into the active component trichloroethanol (TCE) by alcohol dehydrogenase. The plasma level peaks at 30 to 60 minutes. TCE is 45% protein bound. TCE undergoes glucuronidation with some oxidation to trichloroacetate (TCA). The half-life of TCE is 8 to 12 hours, whereas that of TCA is 67 hours. In infants and neonates, this may be increased by a magnitude of three to four. With multiple dosing, a significant potential exists for accumulation. TCA can displace bound bilirubin from albumin. Its actions include CNS depression with drowsiness and sleep in less than an hour. With an overdose, the patient falls into a deep stupor or coma, and the pupils change from constricted to dilated. At therapeutic levels, the blood pressure and respiratory rate are unaffected.
Chloral hydrate has little hangover effect. It has several effects on the cardiovascular system, including decreased myocardial contractility, a shortened refractory period, and an increased sensitivity of the heart to catecholamines. It also has effects on mucous membranes. Irritation can cause gastritis, nausea, and vomiting. With overdose, a severe hemorrhagic gastritis with gastric necrosis and esophagitis has been described. Chloral hydrate and ethanol interfere with one another’s metabolism through competition for alcohol dehydrogenase. Also, ethanol inhibits the conjugation of TCE and TCE inhibits the oxidation of ethanol. Coumadin activity may be increased by chloral hydrate. Chloral hydrate is synergistic with other sedative agents. In children receiving amphetamine-based medication, chloral hydrate is contraindicated because there have been rare reports of arrhythmias. The reversal of chloral hydrate with flumazenil has been described; however, a report of ventricular tachycardia with this combination also has been made.
Chloral hydrate is available as capsules, syrup (50 mg/mL), and suppositories. The sedative dose is 25 to 50 mg/kg (PO/by way of the rectum), whereas up to 100 mg/kg can be safely used in children younger than 5 years with a maximum dose of 1 g. Because of an increased half-life, neonatal dosing should be lower (25 mg/kg). In preterm babies, toxicity resulted when chloral hydrate was used for 3 days. In term babies, toxicity resulted when it was used for 7 days. The therapeutic level for TCE is 2 to 12 μg/L; toxicity occurs when the level is more than 25 μg/L. Chloral hydrate provides successful moderate sedation in approximately 90% of patients, but it appears to be less effective in patients older than 2 years. A higher risk of failure with excessive effect can be seen in patients with a history of obstructive sleep apnea or encephalopathy.
A recent meta-analysis showed that the use of chloral hydrate in procedural and diagnostic sedation in children appears to be effective and fairly safe when administered in the appropriate settings with appropriate monitoring. Similar to any other procedural sedation, a thorough preprocedural patient assessment and appropriate patient monitoring—including pulse oximetry and heart rate both during the procedure and during recovery—are highly recommended. However, newer, safer, reversible sedation medications are readily available, with shorter durations of effect that lower the risk for postprocedural oversedation. In a randomized, prospective study of 150 children under the age of 3 years with known or suspected congenital heart disease scheduled for transthoracic echocardiography with sedation, chloral hydrate was as effective as intranasal dexmedetomidine.
Signs of chloral hydrate toxicity are usually noted within 3 hours of dosing. Paradoxical excitement also has been described in 6% of patients. There is some evidence that chloral hydrate may be genotoxic and carcinogenic. Mice studies have shown that a single-dose exposure can result in an increased risk of hepatic carcinomas and adenomas. Chloral hydrate overdose produces a clinical picture that is similar to acute barbiturate poisoning. Ataxia, lethargy, and coma occur within 1 to 2 hours. Also, a pear-like odor may be noted. Cardiovascular instability poses the main threat to life. Severe arrhythmias—including atrial fibrillation, supraventricular tachyarrhythmia, ventricular tachyarrhythmia, torsades de pointes, and ventricular fibrillation—have been described.
Chronic use can cause a dependence syndrome. Also, chloral hydrate is not detectable in the blood. TCE levels are measurable, but they are not useful for clinical management, although they can be helpful for retrospective forensic diagnosis. The management of toxicity includes evaluation and monitoring at a medical facility if an amount greater than 50 mg/kg or an unknown amount has been ingested. Charcoal with intubation should be considered if significant toxicity is suspected. Standard antiarrhythmic management is often unsuccessful, although esmolol, overdrive pacing, and hemoperfusion have been tried.
Butyrophenones and phenothiazines
Haloperidol
Butyrophenones belong to a group of major tranquilizers. Haloperidol is a potent antipsychotic agent with nonspecific dopamine antagonist action. It has little effect on the cardiovascular or respiratory systems. It produces the appearance of calm with minimal hypnotic effect and reduces operant behavior (purposeful movement). The patient appears tranquil and dissociated from surroundings but is readily accessible if spoken to. Haloperidol may mask actual feelings of mental restlessness. It is a potent antiemetic agent (with action at the chemotrigger zone) and has no appreciable effect on the EEG. It potentiates analgesics and other sedative agents. Compared with less potent butyrophenones, it has fewer adverse effects. Neuroleptanalgesia, a dissociative form of anesthesia, can be induced when haloperidol is combined with high-dose opiates. This anesthetic state is useful for certain cardiac and neurosurgical procedures that require cardiovascular stability and a responsive patient. Haloperidol is metabolized to inactive compounds with a half-life of 15 to 25 hours. It is highly protein bound. Hepatic dysfunction increases the half-life because of reduced clearance. Adverse effects include extrapyramidal signs, although acute dystonia is rare. Prolongation of the QT interval is possible, with the attendant risk of ventricular tachycardia. Hepatic toxicity can occur but is rare. Haloperidol is indicated for the treatment of psychoses, Tourette disorder, and severe behavioral problems in children. In the PICU, it is used as a treatment for agitation in patients who are unresponsive to other more commonly used agents. It also has proved to be effective as part of a sedative withdrawal strategy. The usual dosage for agitation in children younger than 3 years is 0.01 to 0.03 mg/kg every 4 hours. The maximum daily dose is 0.15 mg/kg per day. Haloperidol is available as syrup, tablets, and an IM preparation. Two IM preparations are available: lactate is for repeated use and decanoate is a slow-release monthly formulation. Although not approved by the FDA, the IM lactate form has been given intravenously without problems.
Haloperidol has been used to treat pediatric hyperactive delirium in critical care units , once other measures, including managing the underlying critical illness and preventing possible iatrogenic factors (e.g., BZDs, sleep deprivation), have failed to alleviate the symptoms of delirium. IV, oral liquid, oral tablet, and IM formulations/routes are available. A dose of 0.05 mg/kg per day divided twice daily can be used. For urgent use in children less than 3 years of age, a dose of 0.025 mg/kg is used; for children age 3 years or older, a dose of 0.5 to 1.0 mg every 15 to 20 minutes is used until agitation and symptoms of delirium are controlled. A maintenance oral dose of 0.05 to 0.15 mg/kg per day divided every 6 to 12 hours is suggested if needed. IV haloperidol may be considered for use to treat hyperactive delirium or if unable to give enteral medication. It is important to note that IV dosing is twice as potent as enteral; thus, a lower dose must be used.
Droperidol
Droperidol is a dopamine receptor antagonist that causes analgesic, sedative, and antiemetic effects. It is faster acting than haloperidol, with a shorter duration of action and a half-life of 2 hours. It is available as an approved IV formulation. With an IV bolus, mild hypotension occurs because of mild α-adrenergic receptor blockade. Droperidol is more sedating than haloperidol and may be used as an adjunct to general anesthesia. It also is used in low doses (0.05 mg/kg) as an antiemetic agent. Concerns exist about the potential for droperidol to cause prolongation of the QT interval that may result in ventricular arrhythmias, but the QT prolongation can also be transient with no major sequelae.
Recent reports suggest that droperidol can be safely used in children for acute behavioral disturbance as a sedative in the prehospital setting as well as in emergency departments for its analgesic, antiemetic, and sedative effects.
Chlorpromazine
Chlorpromazine is a weaker antipsychotic agent with general CNS depressant activity. It has an antidopaminergic effect—including extrapyramidal adverse effects, lethargy, and apathy—with an EEG similar to that of normal sleep. It also causes a decrease in the body’s ability to maintain temperature control, shivering is reduced, and it can be useful in patients in hypothermic-induced states. Cardiovascular effects include α-adrenergic receptor blockade with hypotension and postural hypotension, but no effect is seen on the ECG. Respiratory drive and depth are unaffected; however, some mucosal dryness may be noted. In the GI tract, its anticholinergic effect causes decreased secretions and motility. Liver effects include jaundice, which occurs in 0.5% (recurrence rate, 40%) independent of dose or duration of therapy. It is also associated with a rash, fever, and eosinophilia. This syndrome has a low mortality rate and usually resolves quickly upon discontinuation of chlorpromazine. Other effects include antihistamine-like action; local analgesia; a temporary leukopenia; and, rarely, agranulocytosis. Chlorpromazine also has antiemetic properties. Indications include premedication, sedation as part of the lytic cocktail catheterization mixture number 3 (CM3), intractable pain, antipsychosis, treatment of hiccoughs, prevention of succinylcholine pain, and induction of hypothermia (with other active measures). Dosing (0.05–1.00 mg/kg every 6 hours) may be via the PO, IM, IV, or rectal routes. Chlorpromazine has been used in critically ill children with delirium that is not responding to standard therapy. A dose of 0.4 mg/kg IV every 4 hours was reported to be safe and effective. Chlorpromazine is metabolized both in the gut wall and by the liver. It yields more than 50 metabolites, most of which are inactive. Other phenothiazine derivatives include prochlorperazine, which has mainly antiemetic properties. Extrapyramidal adverse effects are more common in children younger than 5 years. Dosage is a PO or rectal dose of 0.40 mg/kg every 8 hours and an IM or IV dose of 0.15 mg/kg.
Lytic cocktail
The lytic cocktail CM3 is a mixture of 25 mg/mL of meperidine, 6.5 mg/mL of promethazine, and 6.5 mg/mL of chlorpromazine. Its recommended dose is 0.1 mL/kg of body weight, but significant institutional variations exist. The CM3 was popular as sedation for cardiac catheterization. However, it has been reported to have a high failure rate and lacks several important characteristics of an ideal sedative for children. Dosing cannot be titrated easily and individually. Onset of action is delayed (30 minutes), and duration of effect is protracted (5–20 hours). CM3 has no anxiolytic or amnestic properties. Additional caution should also be exercised when this cocktail is used in children with seizure disorders. The metabolite of meperidine and the lowered seizure threshold from chlorpromazine put the patient at risk. Patients with congenital heart disease with physiologic conditions such as tetralogy of Fallot or left ventricular outflow obstruction may be put at risk because of systemic vasodilation that causes altered blood flow through shunts, a hypercyanotic spell, or decreased coronary blood flow due to diastolic hypotension.
Neuroleptic malignant syndrome
Both the butyrophenones and phenothiazines have a rare but well-described adverse effect called the neuroleptic malignant syndrome. It involves the development of hypertonicity, with autonomic instability, fever, and cognitive disturbance. The incidence is 0.5% to 1.4% of patients exposed to neuroleptic agents. The true incidence in children is unknown, however. Several different diagnostic criteria are available. Fever and rigidity present in all cases; other symptoms are shown in Box 132.3 . A variety of therapies have been described, including supportive treatment and discontinuation of the neuroleptic agent, anticholinergics, amantadine, bromocriptine, dantrolene, L -dopa, and electroconvulsive therapy. Several other rarer diagnoses may manifest this myriad of symptoms, such as paroxysmal sympathetic hyperactivity syndrome following traumatic brain injury, serotonin syndrome, and anti-NMDA receptor encephalitis.
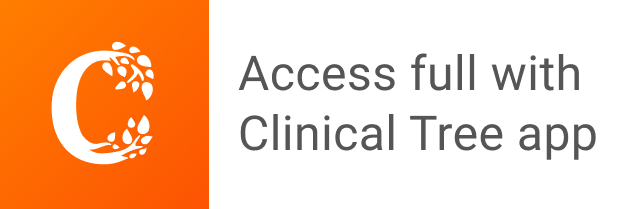