Pearls
- •
The kidney is the primary elimination pathway for the majority of medications. Thus, understanding appropriate dosing for patients in acute and chronic renal impairment is imperative to avoid adverse events and therapeutic failure.
- •
Kidney disease not only affects renal elimination of medications but can also affect absorption, metabolism, and protein binding in acute and chronic kidney disease.
- •
Intravenous fluid therapy to maintain adequate urine output has been shown to be effective for the prevention of acute kidney injury but not for treatment.
- •
Medication dosing in renal replacement therapy is dependent on the medication properties, intrinsic renal clearance, and the renal replacement prescription.
- •
Loop diuretics are dose dependent. More diuresis is achieved at higher doses until the “ceiling dose” is reached.
- •
Continuous infusion of loop diuretics can result in a steadier diuretic effect than intermittent high doses while also avoiding the high and low serum concentrations associated with toxicity and resistance.
The kidney is an important target for drug therapy in the critically ill child and plays a central role in many physiologic processes that have a direct impact on drug action and disposition. Key functions of the kidney include elimination of endogenous and exogenous substances from the body, including drugs and drug metabolites, as well as the maintenance of body fluid composition and volume. There are complex glomerular and tubular mechanisms affecting these key functions that are directly and indirectly influenced by the function of other organs. As one of the primary routes of medication and metabolic waste elimination, the kidneys are highly susceptible to toxicity and insult leading to renal failure. The inability of the kidney to perform such key functions in renal failure may have deleterious effects on the function of other vital organs and physiologic systems. Alteration in kidney function significantly affects drug disposition—including changes in absorption, distribution, metabolism, and elimination. With a significant number of renally cleared medications being used in the intensive care unit (ICU), special attention must be paid to kidney function and the effect of impaired renal function on drug elimination and metabolism in order to avoid medication toxicities or therapeutic failure.
Kidney function and drug disposition
Renal function in neonates, infants, and children is affected by a multitude of factors, including—but not limited to—development and maturation, underlying kidney disease and comorbidities, medications, and renal replacement therapy (RRT). Neonates have decreased renal function at birth, with the glomerular filtration rate (GFR) increasing to 20 to 30 mL/min per m 2 in term neonates at approximately 2 weeks of life and reaching adult levels at about 6 months of age. Critically ill pediatric patients are at an increased risk of altered renal function. Thus, it is important to understand how renal failure affects medication pharmacokinetics. Renal elimination of medications is achieved through glomerular filtration, tubular secretion, reabsorption, and characterization of transporters on the renal tubule epithelium. GFR is estimated with the measurement of the rate that the kidney removes a substance from the blood (i.e., renal clearance). For drugs and drug metabolites that are primarily eliminated by glomerular filtration, such as gentamicin, the rate of elimination mirrors kidney function. As such, when kidney function declines, the reduced drug elimination results in drug accumulation in the body ( Fig. 77.1 ). Decreased GFR is a hallmark feature of both chronic kidney disease (CKD) and acute kidney injury (AKI); it is important to identify the most accurate measurement of GFR in pediatric patients to appropriately adjust medication dosing (see Chapter 71 ).

Although GFR is the most commonly used marker of renal function for medication dosing, renal transporters also play an important role in elimination of drugs and their metabolites. Albumin and other large molecules do not pass through the glomeruli. Thus, in general, drugs with high molecular weight and drugs bound to plasma proteins (e.g., furosemide) are not effectively removed by glomerular filtration but may be efficiently eliminated by the kidney through renal tubular secretion. Active transport of drugs cleared by secretion or reabsorption (e.g., ganciclovir and famotidine) also decreases in acute and chronic renal impairment. It is important to assess dosing of medications that are eliminated not only through filtration but also through secretion or reabsorption in patients with impaired renal function. ,
Decreased renal elimination of drugs and drug metabolites is the most obvious consequence of altered renal function. However, renal failure and associated coexisting conditions may also affect drug absorption, distribution, and metabolism ( Table 77.1 ). Delayed gastric emptying, changes in gut motility, and modifications in gastric pH found in patients with renal disease can alter absorption and bioavailability of drugs. CKD has been shown to decrease plasma protein binding due to hypoalbuminemia, leading to increased free unbound fraction of drug and, ultimately, increasing patients’ drug exposure. Hydrophilic drugs may be affected in patients in renal failure due to edema, increased total body water, and an increase in volume of distribution—thus, decreasing drug exposure. Renal failure is also associated with decreased activity of hepatic and gastrointestinal drug-metabolizing enzymes and transporters, leading to decreased clearance of nonrenally eliminated drugs. Considering that renal failure can influence medication distribution in a multitude of ways, it is important to review all medications, including those not cleared renally, for safety and efficacy in pediatric patients with renal failure.
PK Parameter | Effect | Proposed Mechanism |
---|---|---|
Absorption | ↓ | Edema of GI tract, uremic N/V, delayed gastric emptying Drug interaction: phosphate binders, H 2 blockers Altered GI pH |
Distribution | ↑ | Increased unbound drug fraction Hypoalbuminemia (nephrosis, malnutrition) Uremic changes in albumin structure |
Metabolism | ↓ ↑ | Inhibition of CYP-450 metabolism (liver, intestine, kidney) Drug interaction Direct inhibition by “uremic” milieu Induced CYP-450 metabolism |
Excretion | ↓ | Decreased GFR Decreased tubular secretion Increased tubular reabsorption |
Drug dosing in kidney disease
Determining safe and effective individualized dosing regimens for critically ill children begins with an estimation of the child’s kidney function with measurement of serum creatinine, calculating the eGFR, and monitoring urine output. The next step is to evaluate the effect of kidney failure on the drug disposition characteristics for all of the drugs prescribed to the child. A systematic approach to individualized drug therapy in children with kidney failure will maximize therapeutic efficacy and minimize toxicity ( Box 77.1 ).
- 1.
Estimate the glomerular filtration rate.
- 2.
Determine the percentage of drug eliminated by the kidney.
- 3.
Adjust the medication dose or frequency of the dosing interval.
- 4.
Monitor therapeutic response and adverse reactions.
- 5.
Perform therapeutic drug monitoring when available.
Available literature for drug dosing in pediatric kidney failure is limited; thus, the majority of dose recommendations are extrapolated from adult literature. Tertiary drug dosing references—such as Lexicomp , Micromedex , and Drug Prescribing in Renal Failure —can be a starting point to determine the optimal dose for patients . Due to pediatric and critically ill patients’ changes in pharmacokinetics and pharmacodynamics, critically ill children with impaired kidney function are at an increased risk of under- or overdosing medications. , It is important to recognize that pharmacokinetics and pharmacodynamics are different in patients with CKD versus those with AKI. Patients with AKI may not show renal failure through endogenous biomarkers during the initial stages. Thus, other signs of AKI are important to assess (e.g., urine output and organ perfusion). Endogenous biomarkers such as serum creatinine and cystatin C are good biomarkers when at steady state, such as in CKD. However, they are not as reliable in AKI when renal function is dynamic (see also Chapter 71 ).
When drugs are adjusted for renal impairment, they should always be adjusted based on renal and nonrenal clearance of the drug, the degree of renal impairment, and the potential nephrotoxicity risk of the drug. The development of drug dosage adjustments for patients in CKD is based on the desired exposure goal at steady state for the specific medication. Certain drugs are dosed based on a goal to keep an average steady state of drug concentration above the minimum inhibitory concentration (MIC; e.g., cephalosporin antibiotics) and others are dosed based on the relationship of peak drug concentration to effect (e.g., gentamicin). , In medications that are dependent on time above MIC, the dose may be decreased but dosing frequency kept the same depending on the degree of renal failure. For medications that are dependent on peak drug concentration effect, the dose may stay similar but the dosing interval will be lengthened to keep the peak concentration similar and avoid accumulation. Therapeutic drug monitoring should be used when able to ensure therapeutic efficacy and decrease toxicity. For example, when assessing gentamicin dosing, the dosing interval is increased and the size of the drug dose remains unchanged ( Fig. 77.2 , dashed line ); the steady-state peak and trough drug concentration are similar to those seen in children with normal renal function. However, there is a prolonged period when the serum gentamicin concentration is above and below the average steady-state concentration. This dosing regimen may be inappropriate for drugs that should be maintained at a relatively stable serum concentration, such as cephalosporins or antihypertensive medications. When the goal is maintenance of a serum concentration close to the steady-state level throughout the dosing interval, decreasing the size of the dose while maintaining the normal dosing interval will decrease the variation between the serum drug concentration peak and trough (see Fig. 77.2 , solid line ).

Critically ill children with AKI pose different challenges to medication dosing. Patients with AKI commonly have an increase in volume of distribution (Vd) due to a positive fluid balance in the initial stages. Knowing whether the drug is hydrophilic or lipophilic and knowing the drug-specific Vd is important to understanding whether a loading dose may be required. Hydrophilic drugs have a higher Vd in patients who are fluid positive in the early stages of AKI and thus may require higher initial dosing but will eventually have slower clearance. Protein binding can also be variable in patients with renal dysfunction; thus, it is important to know whether a medication is highly protein bound. For medications that are highly protein bound, patients will have an increase in unbound or free drug due to decreased albumin binding, leading to a higher overall exposure to the medication. Critically ill pediatric patients in renal failure are at increased risk of therapeutic failure or medication toxicity. Therefore, close attention to patient clinical status and medication pharmacokinetics is essential when dosing medications.
Drug dosing in dialysis
RRT is used in approximately 5% of pediatric intensive care unit (PICU) patients. Predominant diagnoses necessitating RRT are AKI, electrolyte abnormalities, volume overload, and toxin removal. , The most common modality of RRT used in the ICU setting is continuous renal replacement therapy (CRRT), followed by intermittent hemodialysis (IHD), and, to a lesser extent, peritoneal dialysis (PD). Refer to Chapter 75 for more complete information on dialysis modalities and indications. Drug elimination in children receiving dialysis is a composite of nonrenal drug elimination, residual kidney elimination, and extracorporeal removal by dialysis. Efficiency of a given dialysis modality to eliminate a drug depends on the physiochemical characteristics of the drug and the form and characteristics of the dialysis procedure. Less than 20% of medications frequently used in children requiring CRRT have dosing guidance across all age groups. Most dosing is extrapolated from adult literature or studied in select age groups in the pediatric population. In general, highly protein-bound drugs and drugs with a large Vd are not removed well by dialysis.
There are three primary types of CRRT used in the ICU: continuous venovenous hemofiltration (CVVH), continuous venovenous hemodialysis (CVVHD), and continuous venovenous hemodiafiltration (CVVHDF). It is important to distinguish among CRRT therapies, as the effect on medication clearance varies between each type. CVVH relies on convection to remove medications, allowing clearance of larger drug molecules (≤30,000 Da). CVVHD uses diffusion to clear medications, which only allows for clearance of small drug molecules (≤500 Da). CVVHDF uses a combination of both convection and diffusion. Correct dosing of medications in ICU patients on CRRT can be extremely challenging due to the combination of altered pharmacokinetics of critical illness, the dynamic nature of a CRRT prescription, and the variability found in medication dosing resources.
Many tertiary medication dosing resources do not specify the CRRT modality in their dosing recommendation and instead use the umbrella term CRRT . These resources do not account for the modality-specific differences in drug clearance, range of dialysis prescription, or intrinsic renal clearance of the patient. With sepsis being a major reason for AKI in the ICU, many recent studies have highlighted the risks of underdosing antimicrobials when using tertiary medication dosing resources. , , , One study found subtherapeutic antibiotic dosing rates as high as 60%. For example, per Pediatric Lexicomp , CRRT dosing for cefepime is 50 mg/kg per dose every 12 hours regardless of age. Recently, Stitt et al. published a small study of four pediatric patients receiving CVVHDF who received cefepime monitoring levels. The cefepime dose was fairly consistent around 50 mg/kg per dose but the frequency of administration varied from every 6 hours to every 12 hours. Only one patient in their study reached the pharmacodynamic goal of percent of free drug greater than 4 times the minimum inhibitory concentration (% fT > 4 × MIC). This study highlights the variability of CRRT prescriptions on medication clearance and, while this study was not large enough to recommend a clear dosing regimen, it also highlights the importance of individualizing drug dosing based on the pharmacokinetic and pharmacodynamic properties of the medication as well as the dialysis prescription.
In order to accurately dose a medication in CRRT, a practitioner must understand the physicochemical characteristics of molecular weight, protein binding (≥80% less likely to be cleared), charge (cationic drugs less likely to be cleared), Vd (Vd >0.7 L/kg less likely to be cleared), and water solubility (low-water-solubility drugs are less likely to be cleared). When dosing antibiotics, one must also understand the pattern of bactericidal activity and presence/duration of postantibiotic effect. For example, an antibiotic with a low Vd, a molecular weight of 547 Da, low protein binding, and high water solubility is going to be readily cleared by convective modes of CRRT but not by diffusive modes. If the bactericidal activity of this antibiotic is based on time above MIC, with no postantibiotic effect, a continuous infusion or frequent intermittent administration may be required to adequately treat an infection on CVVH or CVVHDF.
Another dosing strategy used in CRRT uses the sieving coefficient (S c ). Many studies have looked at the S c of the drug to help understand how freely it crosses the dialysis membrane.
An S c of 1 indicates free removal across the dialysis membrane, while an S c of 0 means no removal. The S c of a medication can be found only through study data and is therefore not available for all medications. However, the S c can be estimated by assuming that the S c is equal to the free fraction of drug in the plasma. Using the S c , the clearance of drug can be estimated by the specific CRRT modality. Example: CVVH clearance estimation = (flow rate of ultrafiltrate) × (S c ).
Many of the same properties that influence medication dosing in CRRT hold true for IHD. Medication clearance in IHD is more impacted by Vd because medications with a high Vd are more difficult to remove in IHD. In CRRT, there is a constant re-equilibration of drug between the tissues and plasma allowing increased medication clearance. In contrast, with IHD, the rate of extracorporeal drug removal from the plasma exceeds the re-equilibration from the tissues. This leads to a posttreatment rebound of plasma concentrations that has to be taken into account when evaluating post-IHD drug levels. There have been multiple advances in IHD equipment over the past 20 years; thus, older studies regarding medication removal in IHD may not be applicable to current practice. High-flux dialysis filters are now routinely used, which allows for clearance of molecules of 20,000 Da or less. IHD medication clearance is highly reliant on IHD time, volume removed, IHD frequency (e.g., daily vs. every other day), and the previously mentioned physiochemical properties of the drug. Similar to CRRT dosing, tertiary drug dosing resources assume that the patient is anuric and that the entirety of clearance depends on dialysis, which is not always applicable to the patient situation. Consideration of administering medications cleared by IHD after the dialysis session should be routinely done or supplemental doses should be used after each dialysis session. In general, most patients need less frequent dosing of renally cleared medications while on IHD due to the significantly reduced or lack of clearance between IHD sessions. Some centers are starting to use a variation of IHD referred to as slow low-efficiency dialysis (SLED) or slow extended daily dialysis, which uses the same IHD equipment but runs the session over 6 to 10 hours. There is very little information available for medication dosing in this modality. Caution should be taken to not overdose or underdose medications in this situation, and drug monitoring should be used when possible.
PD is the most inefficient form of dialysis for medication removal. A typical PD prescription targets a clearance of urea close to 10 mL/min. Since almost all drug molecules are larger than urea, medication clearance would be expected to be low. Due to this level of clearance, patients on PD are at higher risk of drug accumulation and should have level monitoring when possible.
Overall, medication dosing in RRT is a very complex and dynamic situation. Initial or loading doses in patients requiring RRT rarely need to be adjusted. However, subsequent dosing needs to be evaluated based on expected total patient clearance (intrinsic clearance + dialysis clearance). , Weighing the risks of underdosing with the risks of overdosing may help guide decision-making while monitoring for medication side effects or disease progression. , When available, obtaining drug levels should be the gold standard of monitoring while on any form of RRT, especially for narrow therapeutic window medications.
Kidney as a therapeutic target: Diuretics and agents regulating renal excretion
Diuretics and agents regulating renal excretion are a diverse group of drugs that act on the kidney to increase salt or water excretion. In the intensive care setting, these drugs are commonly prescribed for mobilization of excess body fluid by promoting diuresis, which, in turn, decreases extracellular fluid volume. They are used in the treatment of arterial hypertension, electrolyte imbalances, and edematous states, such as cerebral, pulmonary, or general edema; ascites; hepatic cirrhosis; and congestive heart failure (CHF). Less common indications include disorders of calcium metabolism, glaucoma, nephrogenic diabetes insipidus, and drug overdoses.
Renal tubular cells transport solute and water from the apical cell membrane to the basolateral cell membrane. Reabsorption of sodium is central to the kidney’s ability to reabsorb water and other solutes (e.g., glucose, amino acids, bicarbonate). Apical cell sodium entry is mediated by channels that permit sodium to enter by diffusion or transport by specific proteins located on the apical cell membrane. In all renal tubular cells, the sodium-potassium adenosine triphosphatase located on the basolateral membrane maintains the low intracellular sodium concentration that favors sodium movement from the tubular fluid into the renal tubular cell. Diuretics inhibit sodium reabsorption by blocking sodium channels or sodium transport proteins located on the apical cell membrane at discrete sites along the nephron. Diuresis is associated with a decrease in vascular volume that stimulates movement of sodium and water from the interstitial space into the vascular space as well as stimulation of counterregulatory pathways that serve to maintain an adequate extracellular fluid volume.
The response to diuretics is determined by the amount and time course of drug reaching the site of action and sensitivity of the active site to the diuretic. There exists a therapeutic threshold (minimal concentration) that must be reached at the site of action before any response is noted and a maximal response (ceiling) above which no further response will occur even if more drug reaches the site of action. The amount and time course of drug reaching the site of action, and tubular response to the diuretic, are influenced by the route and frequency of administration as well as drug and disease states that modify the amount of diuretic reaching the tubular fluid. An example is the twofold to threefold increase in loop diuretic dosage required for response in patients with decreased kidney function. In kidney failure, the entry of loop diuretics into the tubular fluid is limited by decreased renal blood flow and competitive inhibition of diuretic transport by “uremic toxins.” Adequate tubular fluid diuretic concentration and response can be achieved with the administration of high doses of loop diuretic.
Carbonic anhydrase inhibitors
Mechanism and sites of action
Carbonic anhydrase (CA) enzymes are located at the brush border membrane of the proximal tubule cells and inside of all proximal tubule cells. CA catalyzes the dehydration of carbonic acid to water and carbon dioxide and inhibition of CA in these sites, leading to a decrease in hydrogen ion formation and a subsequent decrease in sodium transport from the lumen into proximal tubule cells. This results in an increase of sodium in the tubular fluid and a decrease in HCO 3 reabsorption.
Efficacy on urinary excretion and therapeutic uses
CA inhibitors are generally considered to be weak diuretics. Their diuretic efficacy is impeded by sodium reabsorption downstream at several nephron sites.
Acetazolamide, the most commonly used CA inhibitor, is more clinically useful for its extrarenal effects than its diuretic effects. Clinically, acetazolamide is used to treat metabolic alkalosis caused by loop diuretics, glaucoma, acute mountain sickness, and occasionally epilepsy. However, its long-term use is limited by the metabolic acidosis that develops because of the bicarbonate loss in the urine.
Adverse effects
In addition to metabolic acidosis, adverse effects of acetazolamide include paresthesia, drowsiness, and renal calculi.
Osmotic diuretics
Mechanism and sites of action
Osmotic diuretics are freely filtered at the glomerulus. They act on both the proximal tubule and on the loop of Henle (primary site of action) and function as solutes in water, increasing tubular osmolality. This, in turn, promotes water retention in tubular fluid, expanding extracellular fluid and increasing renal blood flow. Ultimately, these processes increase urine volume.
Efficacy on urinary excretion and therapeutic uses
Osmotic diuretics increase urinary excretion of most electrolytes (Na + , K + , Ca 2 + , Mg 2 + , Cl – , HCO 3 , and phosphate). Mannitol, a sugar alcohol, is the prototypical osmotic diuretic. Extraction of water from the intracellular compartments to the extracellular fluid volume that is associated with mannitol administration is clinically useful in cerebral edema, glaucoma, management of drug toxicities, and the prevention of dialysis disequilibrium syndrome.
Adverse effects
Mannitol-induced expansion of extracellular fluid volume may be sufficient to perpetuate CHF, pulmonary edema, and significant hyponatremia in patients with renal failure in whom the half-life of mannitol is prolonged and the ability to excrete free water is limited.
Loop diuretics
Mechanism and site of action
Loop diuretics decrease sodium reabsorption by inhibiting the electroneutral Na + /K + /2Cl − cotransporter located on the apical cell membrane in the ascending limb of Henle. This inhibition increases distal tubular concentration of sodium and reduces water reabsorption by increasing the tubular fluid osmotic force and reduction of hypertonicity of medullary interstitium.
Pharmacokinetics
Loop diuretics display similar efficacy but differ slightly in pharmacokinetic characteristics. Bumetanide and torsemide are almost completely absorbed after oral administration, whereas the absorption of furosemide is extremely variable, with an average bioavailability of 50% in adults and up to 84% in neonates. , The onset of diuretic effect is within minutes of intravenous (IV) administration of a loop diuretic and 30 to 60 minutes after oral administration. The duration of diuretic effect is short (2–6 hours); this short duration often results in the need for multiple doses or a continuous infusion to achieve the desired effect ( Table 77.2 ). ,
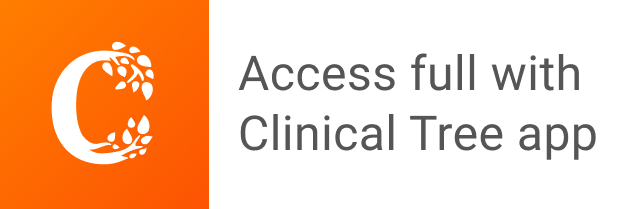