Pearls
- •
When delivering critical care, one must understand the specific properties that characterize the various regional circulations because therapies that benefit one region may be detrimental to another.
- •
Vascular tone is influenced by (1) innervation and neural processes, (2) circulating endocrine and neuroendocrine mediators, (3) local metabolic products, (4) blood gas composition, (5) endothelial-derived factors, and (6) myogenic processes.
- •
The transition from the fetal pulmonary circulation to the postnatal pulmonary circulation is marked by a dramatic fall in pulmonary vascular resistance and rise in pulmonary blood flow. The failure to successfully make this transition is integral to a number of neonatal and infant diseases.
- •
An important feature unique to the cerebral circulation is the presence of a blood-brain barrier. As a result, the cerebral vasculature responds differently from other vascular beds to humoral stimuli.
- •
Regulation of myocardial perfusion is tailored to match regional myocardial oxygen supply to demand over the widest possible range of cardiac workload. Increases in myocardial oxygen demand must be met by increases in myocardial blood flow.
- •
Critically ill patients are at risk for impaired splanchnic blood flow that can impair the two chief functions of the gastrointestinal system: (1) digestion and absorption of nutrients and (2) maintenance of a barrier to the translocation of enteric antigens. Splanchnic ischemia is associated with increased morbidity and mortality in critically ill patients.
- •
Although renal blood flow remains constant over a wide range of renal artery perfusion pressures, urinary flow rate varies as a function of renal perfusion pressure.
General features
General anatomy
Blood vessels comprise several distinct layers. Moving from the innermost layer outward are the metabolically active endothelium, intima (with nerves and vasa vasorum), media, and adventitia. Some vessels have fewer layers depending on the position and function of the vessel within the circulation.
The large arteries are elastic. Their media contain concentric lamellae of perforated elastic tubes crosslinked by transverse collagen and smooth muscle. When smooth muscle contracts, the wall becomes stiffer. Smaller arteries have fewer lamellae. The media are bounded by the internal and external elastic laminae. Arterioles are less elastic, have no lamellae, and have a thin medium with circular or spiral smooth muscle and inner and outer elastic laminae. Capillaries are thin walled and nonmuscular, ideal for transport of materials to and from the tissues. Veins have medial muscle but thinner walls relative to lumen diameter compared with arteries. The vascular endothelium has important metabolic characteristics, which may differ between vessel types (i.e., arteries vs. veins) and different regions. ,
Basic physiology
Blood flow to a regional vascular bed is determined primarily by inflow pressure, vascular resistance, and outflow pressure. Inflow pressure is usually systemic arterial pressure. Outflow pressure approximates venous pressure but may exceed venous pressure at times if vascular tone is great enough to close the circulation above venous pressure or if external pressure impinges on the vasculature.
In a model to explain the relation of arterial pressure to flow, the circulation is represented by two capacitance vessels separated by a resistance. A standpipe full of blood is allowed to discharge its contents into the arterial vasculature (the proximal capacitance). Blood flows across the resistance site (the arterioles), traverses the venous vasculature (the distal capacitance), and drains to a reservoir at some outflow pressure (Po; Fig. 24.1 ).

The pressure head of the system (Pi) is generated by the weight of the column of blood in the standpipe and is proportional to its height (Pi = blood column height in cm H 2 O). As the standpipe discharges, the height decreases and Pi decreases. This, in turn, decreases the rate of flow (Q) through the vasculature. Q decreases almost linearly with Pi until the column is quite low. Ultimately, flow will cease while there is still pressure in the standpipe. The pressure at which flow ceases is the critical closing pressure of the circulation (Pc). Pi below Pc is insufficient to maintain vessel patency and permit continued flow ( Fig. 24.2 ).

Incremental resistance to flow is generally defined as the change in pressure per unit change in flow (dPi/dQ). At pressures well above critical closing pressure, this is nearly identical to the vascular resistance (R) defined clinically as
R=(Pi-Po)/Q
When Pi does not greatly exceed Pc but Pc does greatly exceed Po, however, incremental resistance can differ substantially from this clinical estimate. Thus, an increase in Pc can be confused with a true increase in incremental resistance. For example, a diagnosis of intrinsic pulmonary vascular disease (e.g., pulmonary arterial hypertension) based on measured pulmonary artery pressures in a patient receiving mechanical ventilation with high airway pressures (that raise Pc) may be spurious.
Venous return and cardiac output
A second model illustrates the relationship of cardiac output to intrinsic mechanical properties of the systemic vasculature. In this model, the heart acts as a roller pump, creating a circulation much like that achieved during venoarterial extracorporeal support ( Fig. 24.3 ).

The roller pump displaces blood from the veins to the arteries and then across the resistance imposed by the arterioles. As Q increases, more blood resides in the arteries and less in the veins. This partitioning of blood depends on arterial capacitance (Ca), venous capacitance (Cv), and resistance to flow. At maximal Q, Pi and arterial blood volume are high and venous pressure (Pv) is low. When Pv reaches Pc, the roller pump cannot be increased further because the veins will collapse when Pv is less than Pc.
As Q is reduced, by turning down the roller pump, arterial pressure (Pi) and volume fall and Pv increases. As Q approaches zero, venous pressure approaches the mean circulatory pressure (Pm; Fig. 24.4 ). The importance of this model is that it can be used to illustrate the role of venous return as an independent determinant of cardiac output.

The Starling curve that describes the relationship between preload and contractility (and, hence, cardiac output) is illustrated in Fig. 24.5 . Over the steep portion of the curve, optimization of myocardial preload increases ventricular stroke volume. This curve (see Chapter 23 ) can be superimposed on the venous return curve. Cardiac output occurs at the theoretical intersection of these two curves, representing a given state of cardiac function (Starling curve) and simultaneous set of vascular characteristics (venous return curve; see Fig. 24.5 ).

It is important to recognize that the venous return curve is influenced by changes in blood volume and vascular tone. Transfusion elevates the maximal venous return and thus cardiac output that can be achieved before the system reaches Pc. Hemorrhage has the opposite effect. Because neither transfusion nor hemorrhage directly alters vascular tone, the slope of the curve is not altered ( Fig. 24.6 ). Both interventions alter mean circulatory pressure because they change blood volume.

Changes in vascular tone may alter the maximum venous return (and thus cardiac output) attainable before venous collapse (Pc) at any given intravascular volume. At zero flow, the mean pressure in the system would relate most directly to the volume of blood within the vessels. Thus, changes in vascular tone change the slope of the venous return curve (see Fig. 24.6 ).
In clinical practice, it is unusual for any of these changes in vascular mechanics to occur in isolation. For instance, arteriolar dilation and dilation of other capacitance vessels often occur together. Arteriolar dilation and dilation of capacitance vessels have opposite effects on the venous return curve and, consequently, different effects on cardiac output. It is for this reason that vascular volume expansion is often required in combination with nitroprusside or milrinone infusions in order to ensure adequacy of cardiac output despite a reduction in afterload.
In patients with sepsis, intravascular volume, venous capacitance, vascular resistance, and the inotropic state of the heart can all profoundly influence cardiac output. Descriptions of shock as “warm” or “cold” relate directly to the interaction of these factors. For example, patients with warm shock often demonstrate adequate contractility with low vascular tone, whereas patients with cold shock may have poor contractility with increased or decreased vascular tone. As such, assessments of intravascular volume are important to help guide management.
Critical closing pressure
In many organs as inflow pressure is lowered, Q decreases and ceases at a pressure—the critical closing pressure (Pc)—that is higher than venous pressure. The probable mechanism is the vascular waterfall or Starling resistor. In 1910, Jerusalem and Starling described a device designed to control afterload to the left ventricle and that made possible the study of cardiac contractility. The device consisted of a collapsible rubber tube traversing a pressurized glass chamber ( Fig. 24.7 ). When pressure surrounding the rubber tube exceeded the outflow pressure set by the reservoir, surrounding pressure opposed the flow of blood and became the true outflow pressure of the device. The physiologic counterpart of this occurs in small vessels surrounded by tissue pressure. In the heart, for example, a Starling resistor effect occurs in extramyocardial coronary veins, although there is also evidence for critical closure of small arterioles. No one has yet demonstrated vessel closure directly. However, this might not be necessary because, as small vessels narrow when they are compressed, the wall becomes convoluted and blood cells might become obstructed by the folds even when externally the vessel does not appear to be closed.

Autoregulation
In all organs, when inflow pressure is suddenly raised or lowered while oxygen (O 2 ) consumption remains constant, flow rises or falls transiently but then returns to its former value; the phenomenon is termed autoregulation. Several mechanisms, particularly related to O 2 sensing, have been implicated in this response, but the precise mechanisms are likely complex and multifactorial. For example, studies have described a role for nitric oxide (NO) carried to the tissues by hemoglobin in the form of S-nitrosohemoglobin. Other locally produced gases, such as hydrogen sulfide and carbon monoxide, , may also play a role. Importantly, some autoregulatory mechanisms are specific to individual microcirculations (e.g., macula densa signaling in the renal circulation).
Distensibility and compliance
The distensibility of a vessel is defined as the change in volume as a proportion of the initial volume for a given change in pressure:
Distensibility=ΔVΔP×1V
where V is volume and P is pressure. Veins are much thinner than arteries and are about eight times more distensible. Multiplying distensibility by volume yields DV/DP, which is the definition of compliance. Because venous volume is usually more than three times arterial volume, venous compliance is about 20- to 30-fold greater than arterial compliance. As a result, whenever fluids are infused, the veins accommodate the bulk of the fluid volume.
Vascular resistance
Under normal circumstances, vascular resistance is the major control of organ flow and can be understood by considering the resistance of a Newtonian liquid passing through a rigid tube as defined by the Hagen-Poiseuille equation:
R=(8π)(1r4)η
where R is resistance, l is tube length, r is the internal radius of the tube, and η is the fluid viscosity. Blood is not a Newtonian fluid, but this fact does not affect the accuracy of calculated vascular resistance much. However, vascular beds do contain many “tubes” in parallel. Thus, for vascular systems, a factor k is added that represents the number of vessels. The equation then becomes
R=(8π)(1kr4)η
Because the length and number of vessels and blood viscosity are relatively constant at any one time, change in vessel radius is the major factor responsible for a dynamic change in vascular resistance. Because of the fourth power factor, small changes in radius cause large changes in resistance. Vessel radius is influenced by vascular elasticity and transmural pressure but is mainly regulated by changes in vessel wall smooth muscle tone.
Vascular impedance
Resistance is strictly a steady-state concept. In a pulsatile system, the factors affecting the relationship of pressure dissipation to flow are resistance due to friction and viscosity, fluid inertia, and vessel wall compliance, which combine to produce an impedance to flow that varies with frequency. At zero frequency, steady-state resistance is approximated by the change in mean pressure overflow, but there are substantial contributions made by the first three harmonics that are ignored by this calculation.
Local regulatory mechanisms
Regions of the circulation may differ markedly in their patterns of vascular regulation. A regulatory stimulus can have multiple effects that differ from one location to another. An agent that potently regulates vascular resistance in one region of the circulation may have no effect in another. For example, during hemorrhagic shock, flow is preserved to the heart and brain at the expense of muscle, the kidneys, and the gut.
Vascular tone is strongly influenced by several mechanisms: (1) innervation and neural processes, (2) circulating endocrine and neuroendocrine mediators, (3) local metabolic products, (4) blood gas composition, (5) endothelial-derived factors, and (6) myogenic processes.
Innervation and neural processes
Receptors responsive to neural products (e.g., norepinephrine and acetylcholine) are found throughout the circulation. Nevertheless, innervation and receptor distribution are organ specific, allowing rapid patterned, coordinated redistribution of blood flow and an orchestrated response to events, such as hypoxia, changes in posture, and hemorrhage. Although these receptors respond to circulating agonists (including adrenal epinephrine), as well as to those liberated locally, they are generally associated with innervation by autonomic nerves. In general, presynaptic α-adrenergic stimulation causes norepinephrine reuptake, whereas postsynaptic α-adrenergic stimulation causes norepinephrine release and vasoconstriction. β-Adrenergic stimulation generally causes vasodilation. Cholinergic stimulation (whether sympathetic or parasympathetic) generally causes vasodilation (see Chapters 31 and 123 ).
Circulating endocrine and neuroendocrine mediators
Humoral regulators of vascular tone include angiotensin, arginine vasopressin, bradykinin, histamine, and serotonin. Of less certain significance are aldosterone, thyroxine, antinatriuretic peptide, and various reproductive hormones. Most of these have both direct effects and secondary effects, which tend to be organ specific or regional in nature. Angiotensin plays a special role in the homeostasis of blood pressure and is produced in hemorrhagic or hypovolemic shock. It causes generalized vasoconstriction in both systemic and pulmonary circulations; however, locally, it stimulates the release of vasodilating prostaglandins in the lungs and kidney. Bradykinin is a potent pulmonary and systemic vasodilator released locally by the action of proteolytic enzymes on kallikrein after tissue injury. Histamine is released by mast cells in response to injury and is also a potent vasodilator in most regions of the circulation, but it causes vasoconstriction in the lung.
Local metabolic products
Local metabolic regulation of vasomotor tone provides an ideal homeostatic mechanism whereby metabolic demand can directly influence perfusion. The precise mechanisms underlying the coupling of blood flow with metabolic activity remain unclear. One theory holds that as the metabolic rate increases, so too does the formation of some vasodilating substance. Thus, the regional vasculature relaxes, allowing more O 2 to be delivered in support of this work. As flow rises, the metabolites are washed out, restoring their concentration to normal. Adenosine, for instance, which accumulates locally when tissue metabolism is high and tissue oxygenation is marginal, causes pronounced vasodilation in the coronary, striated muscle, splanchnic, and cerebral circulations. Another example is potassium, which is released from muscle in response to increased work, ischemia, and hypoxia. Hypokalemia causes vasoconstriction; hyperkalemia, within the physiologic range, causes vasodilation.
An increasing amount of data demonstrate the importance of the local redox state on the regulation of blood flow through the microcirculation. Reactive oxygen species, such as superoxide, hydrogen peroxide, and peroxynitrite, have been shown to influence normal regulatory processes and participate in the pathophysiology of a wide array of cardiovascular disorders. For example, the rapid reaction of NO with the superoxide anion results in the formation of peroxynitrite, a potent oxidant. Although peroxynitrite is known to have cytotoxic properties, under normal conditions peroxynitrite inhibits leukocyte adherence and platelet aggregation without evidence of cellular injury. In disease states, however, peroxynitrite can lead to protein nitration and DNA damage. In addition, elevated levels of superoxide may decrease the bioavailability of NO, leading to abnormal vasomotion.
Blood gas composition
Tissue levels of O 2 and carbon dioxide have been shown to reflect adequacy of perfusion and O 2 delivery. These blood gases are potent determinants of regional blood flow and have effects that differ from one region of the circulation to another. They also have a more general effect mediated by carotid chemoreceptors.
Endothelial-derived factors
The vascular endothelial cells are capable of producing a variety of vasoactive substances, which participate in the regulation of normal vascular tone. These substances, such as NO, carbon monoxide (CO), hydrogen sulfide, and endothelin-1 (ET-1), are capable of producing vascular relaxation and/or constriction, modulating the propensity of the blood to clot, and inducing and/or inhibiting smooth muscle migration and replication ( Fig. 24.8 ). Understanding the role of the vascular endothelium and the factors that it produces in regulating blood flow in health and disease has resulted in several treatment strategies that target, mimic, or augment endothelial processes. Therapies that have been used with variable success include inhaled NO for pulmonary hypertension; L -arginine supplementation for coronary artery disease and the pulmonary vasculopathy of sickle cell disease; phosphodiesterase inhibitors, which prevent the breakdown of cyclic guanosine monophosphate (cGMP) for pulmonary hypertensive disorders; endothelin receptor antagonists for pulmonary hypertensive disorders and vasospasm following subarachnoid hemorrhage; and NO inhibitors for refractory hypotension secondary to sepsis. Indeed, many older therapies used to promote vascular relaxation, such as nitrovasodilators, affect endothelial function.

NO is a labile humoral factor produced by NO synthase from L -arginine in the vascular endothelial cell. NO diffuses into the smooth muscle cell and produces vascular relaxation by increasing concentrations of cGMP via the activation of soluble guanylate cyclase. NO is released in response to a variety of factors, including shear stress (flow) and the binding of certain endothelium-dependent vasodilators (such as acetylcholine, adenosine triphosphate [ATP], and bradykinin) to receptors on the endothelial cell. Basal NO release is an important mediator of both resting pulmonary and systemic vascular tone in the fetus, newborn, and adult, as well as a mediator of the fall in pulmonary vascular resistance normally occurring at the time of birth. Dynamic changes in NO release are fundamental to the regulation of all vascular beds.
CO is a labile humoral factor produced by the action of hemoxygenase on heme in many tissues, including endothelial cells. Hemoxygenase-1 is constitutive and hemoxygenase-2 is inducible. CO interacts with NO, is an independent stimulator of cGMP, relaxes smooth muscle, inhibits its replication, and has powerful antithrombotic and antiinflammatory effects. It is beginning to enter the field of clinical medicine. ,
Hydrogen sulfide is produced in most tissues by a variety of mechanisms and may be the ultimate sensor that is stimulated by O 2 deficit or excess.
ET-1 is a 21 amino acid polypeptide also produced by vascular endothelial cells. The vasoactive properties of ET-1 are complex; studies have shown varying hemodynamic effects on different vascular beds. However, its most striking property is its sustained hypertensive action. In fact, ET-1 is the most potent vasoconstricting agent discovered, with a potency 10 times that of angiotensin II. The hemodynamic effects of ET-1 are mediated by at least two distinctive receptor populations, ETA and ETB. The ETA receptors are located on vascular smooth muscle cells and mediate vasoconstriction, whereas the ETB receptors may be located on endothelial cells and mediate both vasodilation and vasoconstriction. Individual endothelins occur in low levels in the plasma, generally below their vasoactive thresholds. This suggests that they are primarily effective at the local site of release. Even at these levels, they may potentiate the effects of other vasoconstrictors, such as norepinephrine and serotonin. The role of endogenous ET-1 in the regulation of normal vascular tone is presently unclear. Nevertheless, alterations in endothelin-1 have been implicated in the pathophysiology of a number of disease states.
Endothelial-derived hyperpolarizing factor (EDHF), a diffusible substance that causes vascular relaxation by hyperpolarizing the smooth muscle cell, is another important endothelial factor. EDHF has not yet been identified, but current evidence suggests that the action of EDHF is dependent on potassium (K + ) channels (see Fig. 24.8 ). Activation of K + channels in the vascular smooth muscle results in cell membrane hyperpolarization, closure of voltage-dependent calcium (Ca 2 + ) channels, and, ultimately, vasodilation. K + channels are also present in endothelial cells. Activation within the endothelium results in changes in calcium flux and may be important in the release of NO, prostacyclin, and EDHF. K + channel subtypes include ATP-sensitive K + channels, Ca 2 + -dependent K + channels, voltage-dependent K + channels, and inward-rectifier K + channels.
The breakdown of phospholipids within vascular endothelial cells results in the production of the important by-products of arachidonic acid, including prostacyclin (PGI 2 ) and thromboxane (TXA 2 ). PGI 2 activates adenylate cyclase, resulting in increased cyclic adenosine monophosphate (cAMP) production and subsequent vasodilation, whereas TXA 2 results in vasoconstriction via phospholipase C signaling (see Fig. 24.8 ). Other prostaglandins and leukotrienes also have potent vasoactive properties.
Myogenic processes
In 1902, Bayliss described an intrinsic increase in vascular tone in response to elevated intravascular pressure. This myogenic response results in alterations in vascular tone following changes in transmural pressure or stretch. This response is especially important at the arteriolar level and is thought to participate in regional autoregulation. Increases in intravascular pressure and/or stretch result in an increase in arteriolar smooth muscle tone, while decreasing pressures have the reverse effect. The precise mechanisms mediating this response are unclear, but a role for dynamic changes in intracellular Ca 2 + and myosin light-chain phosphorylation has been documented. More recent work has focused on the role of tyrosine phosphorylation pathways, ENaC, transient receptor potential (TRP) channels, K + channels, and alterations in Ca 2 + sensitivity in this response. Moreover, the myogenic response varies between the regional circulations and vessels within a given circulation.
Regional circulations
Pulmonary circulation
Maldevelopment and/or maladaptation of the pulmonary vascular bed are important components of several neonatal and infant disease states (i.e., chronic lung disease, persistent pulmonary hypertension of the newborn, and congenital heart disease). In addition, strategies aimed at altering postnatal pulmonary vascular resistance are commonly used in the management of these patients. Therefore, an understanding of the regulation of postnatal pulmonary vascular tone is important.
The morphologic development of the pulmonary circulation affects the physiologic changes that occur in the perinatal period. In the fetus and neonate, small pulmonary arteries have thicker muscular coats than similarly located arteries in the adult. This muscularity is responsible, in large part, for the pulmonary vascular reactivity and high resistance found in the fetus. Within the first several weeks after birth, the medial smooth muscle involutes, and the thickness of the media of the small pulmonary arteries decreases rapidly and progressively.
Following this perinatal transition, the medial layers of the proximal pulmonary vascular bed are completely encircled by smooth muscle. Moving distally, muscularization becomes incomplete (arranged in a spiral or helix) and disappears completely from the most peripheral arterioles. In these arterioles, an incomplete pericyte layer is found within the endothelial basement membrane. Smooth muscle precursor cells reside in the nonmuscular portions of the partially muscular pulmonary arteries. Under certain conditions, such as hypoxia, these cells may rapidly differentiate into mature smooth muscle cells.
Subsequently, from infancy to adolescence, the arteries undergo progressive peripheral muscularization. In the adult, complete circumferential muscularization extends peripherally such that the majority of small pulmonary arteries are completely muscularized.
Normal fetal circulation
In the fetus, normal gas exchange occurs in the placenta and pulmonary blood flow is low, supplying only nutritional requirements for lung growth and performing some metabolic functions. Pulmonary blood flow in near-term lambs is between 8% and 10% of total output of the heart. Pulmonary blood flow is low despite the dominance of the right ventricle, which, in the fetus, ejects about two-thirds of total cardiac output. Most of the right ventricular output is diverted away from the lungs through the widely patent ductus arteriosus to the descending thoracic aorta, from which a large proportion reaches the placenta through the umbilical circulation for oxygenation. Fetal pulmonary arterial pressure increases with advancing gestation. At term, mean pulmonary arterial pressure is about 50 mm Hg, generally exceeding mean descending aortic pressure by 1 to 2 mm Hg. Pulmonary vascular resistance early in gestation is extremely high relative to that in the infant and adult, probably due to the low number of small arteries. Pulmonary vascular resistance falls progressively during the last half of gestation, new arteries develop, and cross-sectional area increases. However, baseline pulmonary vascular resistance is still much higher than after birth. ,
Changes in the pulmonary circulation at birth
After birth, with initiation of ventilation by the lungs and the subsequent increase in pulmonary and systemic arterial blood O 2 tensions, pulmonary vascular resistance decreases and pulmonary blood flow increases by 8- to 10-fold to match systemic blood flow. This large increase in pulmonary blood flow increases pulmonary venous return to the left atrium, increasing left atrial pressure. Then, the valve of the foramen ovale closes, preventing any significant atrial right-to-left shunting of blood. In addition, the ductus arteriosus constricts and closes functionally within several hours after birth, effectively separating the pulmonary and systemic circulations. Mean pulmonary arterial pressure decreases and, by 24 hours of age, is approximately 50% of mean systemic arterial pressure. Adult values are reached 2 to 6 weeks after birth , ( Fig. 24.9 ).
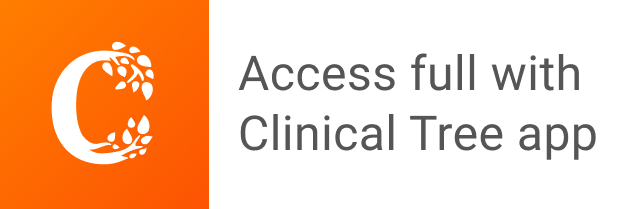