Key Points
- •
Purine nucleotide synthesis and degradation form a crucial metabolic pathway for cell integrity and reproduction.
- •
Phosphoribosyl pyrophosphate synthetase superactivity, a rare X chromosome–linked disorder, causes juvenile gout.
- •
Clinical features of hypoxanthine-guanine phosphoribosyltransferase (HPRT) deficiency include uric acid overproduction–related symptoms, neurologic manifestations, and hematologic disturbances including megaloblastic anemia.
- •
A continuous spectrum of neurologic involvement is present in HPRT-deficient patients.
- •
Compulsive self-injurious behavior is only present in patients with the complete HPRT enzyme defect.
- •
Uric acid overproduction in patients with Lesch-Nyhan disease is effectively controlled with allopurinol.
- •
Xanthinuria is suspected on the basis of a diminished serum urate and urinary uric acid excretion, and most of the patients with classic xanthinuria are asymptomatic.
- •
The 2,8-dihydroxyadenine stones are caused by adenine phosphoribosyltransferase deficiency, a rare autosomal recessive disorder.
- •
The association of hypouricemia, recurrent infections, neurologic symptoms, and autoimmune disease prompts the diagnosis of purine-nucleoside phosphorylase deficiency.
Human Purine Metabolism
Purines are molecules with diverse functions in cell physiology. They are essential compounds for nucleic acid synthesis, energy-requiring reactions, cofactor reactions, and intercellular and intracellular signaling. The pathways for purine synthesis, metabolism, and degradation are shown in Figure 3-1 . Purine metabolism synthesizes the ribonucleotides adenosine monophosphate, inosine monophosphate, xanthosine monophosphate, and guanosine monophosphate (AMP, IMP, XMP, and GMP, respectively), which produce the deoxyribonucleotides, key elements for nucleic acid synthesis (DNA and RNA).

Three different processes yield an adequate cellular purine concentration: de novo purine synthesis from smaller organic molecules, salvage of preformed purine bases, and purine uptake from the extracellular medium.
Purine Synthesis and Regulation
The de novo purine synthesis occurs through a multistep process regulated at different points (see Fig. 3-1 ).
The first step is the synthesis of phosphoribosyl pyrophosphate (PRPP) from ribose-5-phosphate, glycine, and adenosine triphosphate (ATP) (see Fig. 3-1 ). This reaction is catalyzed by the enzyme phosphoribosylpyrophosphate synthetase (PRS). PRPP offers the “phosphoribosyl” skeleton on which several atoms are incorporated through 10 reactions leading to the synthesis of inosinic acid (IMP). The last two reactions are catalyzed by a dual-action enzyme termed AICAR transformylase/IMP cyclohydrolase (ATIC). De novo purine synthesis is mainly regulated by the enzyme amidophosphoribosyltransferase (AMPRT; see Fig. 3-1 ). This enzyme is stimulated by increased substrate concentrations (PRPP) and is subject to feedback inhibition by the purine nucleotides IMP, AMP, and GMP (see Fig. 3-1 , broken lines). Most cells are capable of synthesizing purines de novo, although this is metabolically expensive: the synthesis of one molecule of IMP requires the consumption of four amino acids, two folates, one PRPP molecule, and three ATP molecules.
Purine nucleotide interconversion is also a complex, multistep process, under feedback control. The conversion of IMP to xanthinilic acid (XA or XMP) by the enzyme IMP dehydrogenase (IDH; see Fig. 3-1 ) is the first step leading to guanine nucleotides and is inhibited by GTP. XMP is further converted into GMP via amination by GMP synthetase (GS). Similarly, the conversion of IMP to adeniylsuccinate (AS or S-AMP) by the enzyme adenylsuccinate synthetase (ASS; see Fig. 3-1 ) is the first step leading to adenine nucleotide synthesis (AMP, ADP, ATP; see Fig. 3-1 ). Adenylsuccinate is further converted to AMP by the enzyme adenylsuccinate lyase (ASL, see Fig. 3-1 ). This enzyme catalyzes also the synthesis of AICAR in the de novo purine pathway. The congenital absence of this enzyme causes AS accumulation and a severe neurologic disease. AMP is converted into IMP through the enzyme AMP deaminase (AMPD; see Fig. 3-1 ). Adenylate kinase (ADK) catalyzed the reversible reaction AMP + ATP ↔ 2ADP.
Conditions that increase ATP degradation (i.e., ischemia with hypoxia that limits ATP regeneration from both ADP and AMP) or enhance ATP consumption (i.e., ingestion of ethanol, which is metabolized in the liver to form acetate) promote hyperuricemia. Each mole of ethanol consumes two moles of high-energy phosphate and can increase AMP formation. Most of the AMP formed is used for ATP synthesis (see Fig. 3-1 ), provided an adequate oxygen supply. However, if this is not the case and the increased amount of AMP is not recycled back to ATP, the excessive AMP formed may enter the purine nucleotide degradation pathway, leading to uric acid synthesis. The administration of ethanol has been shown to increase the adenine nucleotide pool turnover (i.e., hyperuricemia associated with alcohol consumption), although lactate production from ethanol metabolism also increases renal urate reabsorption.
Purine nucleotide degradation is initiated by nucleotide dephosphorylation and nucleoside formation (adenosine, inosine, and guanosine) (see Fig. 3-1 ). This reaction is catalyzed by 5′-nucleotidases (5′-NT; see Fig. 3-1 ) and unspecific phosphatases. Inosine and guanosine, through the action of purine-nucleoside phosphorylase (PNP; see Fig. 3-1 ), are transformed into the purine bases hypoxanthine and guanine, respectively. Adenosine is converted into inosine by the enzyme adenosine deaminase (ADA; see Fig. 3-1 ). Finally, the purine bases hypoxanthine and xanthine (oxipurines) are oxidized to uric acid by the enzyme xanthine oxidoreductase (XOR; see Fig. 3-1 ).
XOR was isolated in its xanthine oxidase (XO) form using oxygen as the electron acceptor from cow’s milk, whereas it has been purified in its xanthine dehydrogenase (XDH) form, with NAD + as the electron acceptor. Both enzymes, XO and XDH, are interconvertible products of the same gene. The enzyme originally exists in its XDH form but is readily converted to XO either irreversibly by proteolysis or reversibly by oxidation of Cys residues to form disulfide groups. The reversible or irreversible conversion of XDH to the XO form generates reactive oxygen species (i.e., H 2 O 2 and O 2 – ). The main product of the reversible conversion of XDH to its XO form is O 2 – , and that of XO to its XDH form is H 2 O 2 . Both have a relevant role in a myriad of physiologic and pathologic mechanisms such as reduction of cytochrome c, defense against infectious pathogens, or in the pathology of post ischemia-reperfusion injury.
Guanine, on the other hand, is converted to xanthine by guanine deaminase or guanase (GD; see Fig. 3-1 ). In general, the activity of these enzymes is regulated by substrate availability. In humans and primates, uric acid is the final product of purine metabolism, but in other animals, uric acid is degraded to allantoin by the enzyme uricase (uricase; see Fig. 3-1 ).
Salvage of preformed purine bases: Purine recycling in humans is mediated by three different enzymes: hypoxanthine-guanine phosphoribosyltransferase (HPRT), adenine-phosphoribosyltransferase (APRT), and adenosine kinase (AK; see Fig. 3-1 ). It is estimated that 90% of the free purines generated in the intracellular metabolism are recycled rather than degraded or excreted. This allows for a very efficient functioning with respect to purine metabolism. HPRT recycles hypoxanthine and guanine into IMP and GMP, respectively (see Fig. 3-1 ). Xanthine is also a substrate of HPRT, but the affinity of HPRT for xanthine is much lower than that for hypoxanthine. APRT, structurally and functionally similar to HPRT, recycles free adenine into AMP (see Fig. 3-1 ). HPRT and APRT need the cosubstrate PRPP for purine base recycling. AK (see Fig. 3-1 ) phosphorylates adenosine to generate AMP. AK prevents the diffusion of free adenosine from the cell or its degradation into inosine by adenosine deaminase (ADA; see Fig. 3-1 ). Thus, AK is a very important enzyme that contributes to maintain the purine nucleotide pool.
Maintenance of the purine nucleotide pool is also due to the incorporation of free purine bases and nucleosides from extracellular sources. Dietary vegetables and animal products, through digestion, generate purines following DNA and RNA degradation. These compounds are absorbed from the gut and incorporated into purines by the liver. These purine bases are exported into the bloodstream to be used by other tissues. The salvage enzymes are crucial to incorporate preformed purine bases into the cellular purine pool. Thus, a salvage enzyme deficiency results not only in an inability to salvage intracellular purines but also in an inability to incorporate purines from extracellular sources.
Drugs That Inhibit Purine Nucleotide Degradation
Uric acid is the final compound of purine nucleotide degradation and is synthesized through the enzyme XOR (see Fig. 3-1 ). Uric acid synthesis may be enhanced when cell turnover is increased. This may occur in a myriad of pathologic processes, including enzyme overactivities, proliferative diseases, glycogen storage disease, malignancies, and hematologic disorders. The first drug clinically used to inhibit purine nucleotide degradation was allopurinol. Allopurinol was synthesized in 1950 to inhibit the oxidative degradation of 6-mercaptopurine (an antileukemic agent only active when converted into its cytotoxic nucleotide [thioinosinic acid] by HPRT). Detailed metabolic studies in patients with chronic myeloid leukemia showed that 5% to 7% of a given dose of mercaptopurine was excreted into the urine as the free compound and 25% to 30% was oxidized to the inert thiouric acid. At that time, there seemed to be no feasible way to increase the rate of nucleotide synthesis and attention was given to inhibit the oxidative degradation of 6-mercaptopurine. Among the purine and pyrimidine derivatives studied, 4-hydroxy-(3,4d)-pyrazolopyrimidine (4-HPP or allopurinol) was not only an inhibitor but a substrate for the enzyme XOR that did not affect tumor growth. Allopurinol given concomitantly with 6-mercaptopurine markedly reduced thioinosinic acid and increased 6-mercaptopurine efficacy. The continued administration of allopurinol markedly decreased serum urate concentrations and urinary uric acid excretion.
Since approved in 1964, allopurinol has been a cornerstone in the treatment of hyperuricemic conditions and processes with increased uric acid production, typically identified by increased urinary uric acid excretion. Allopurinol competitively inhibits XOR (see Fig. 3-1 ) and thus reduces uric acid synthesis. In normal conditions, allopurinol increases the reutilization of hypoxanthine for nucleotide and nucleic acid synthesis, via HPRT activity, and thereby consumes substrate (PRPP). The resultant increase in allopurinol nucleotide concentration leads to feedback inhibition of de novo purine synthesis at the level of allosteric regulation of amidotransferase activity.
In patients with HPRT deficiency, allopurinol increases mean xanthine excretion 10-fold and has been associated with xanthine urolithiasis. The long-lived active metabolite of allopurinol is oxypurinol, which normally is predominantly renally cleared and has a 24-hour half-life but its half-life is increased by renal impairment. Oxypurinol is also a competitive inhibitor of XOR (see Fig. 3-1 ). Both allopurinol and oxypurinol are substrates of HPRT. Their corresponding nucleotides exert an inhibitory effect on AMPRT activity and decrease de novo purine synthesis (see Fig. 3-1 ). This explains the fact that the reduction in uric acid synthesis is not replaced by a stoichiometric replacement by hypoxanthine and xanthine.
A new potent inhibitor of XOR has been recently approved for the treatment of gout. Febuxostat (2-[3-cyano-4-isobutoxyphenyl]-4-methyl-5-thiazolecarboxylic acid), unlike allopurinol, is not a purine analogue and inhibits both the oxidized and reduced forms of the enzyme XOR (see Fig. 3-1 ) in a noncompetitive way, by interfering with access of substrate to the active site of the enzyme. This difference has been postulated to account for the higher potency and long-lasting action of febuxostat compared to allopurinol. Importantly, and contrary to allopurinol, febuxostat is not a substrate of purine and pyrimidine metabolic enzymes, such as HPRT or orotate phosphoribosyltransferase, and it does not inhibit purine nucleoside phosphorylase and orotidine-5′-monophosphate decarboxylase (see Fig. 3-1 , green area).
On a milligram orally administered basis, febuxostat inhibitory effect appears to be superior to that of allopurinol (febuxostat 40 mg equals allopurinol 300 mg) and does not require dosage adjustment in patients with mild to moderate renal impairment (creatinine clearance, 30 to 89 ml/min). The precise indications, dosing, effectiveness, and side effect profile of febuxostat in the treatment of hyperuricemia in gout and other conditions are discussed in Chapter 15 .
Disorders of Purine Metabolism: Classification
Most disorders of purine metabolism are expressed by a considerable variation in serum urate concentration and urinary uric acid excretion, since uric acid is the final product of purine metabolism in human beings (see Fig. 3-1 ). A detailed clinical study from a given patient may disclose whether he or she has a congenital or an acquired disease. This chapter will focus on congenital disorders of purine metabolism that cause hyperuricemia and hypouricemia. Purine metabolism congenital diseases may compromise the following enzymes: (1) purine synthesis de novo—PRS, adenylatosuccinate lyase, and ATIC; (2) salvage purine synthesis—HPRT and APRT; and (3) purine interconversion and degradation pathway—XOR, PNP, ADA, adenylate kinase, and myoadenilate deaminase. On the other hand, certain drugs may also inhibit enzymes of the purine nucleotide degradation pathway or may modify renal uric acid excretion. Enzymopathies may be classified into two main groups: overactivity and deficiency, the last being, by far, the most frequent. From a clinical point of view, purine disorders may be classified according to the clinical syndromes they determine: hyperuricemia and gout ( Table 3-1 ), nephrolithiasis, immunodeficiency, anemia, diseases of the peripheral and central nervous systems, and myopathies ( Table 3-2 ).
HYPERURICEMIA AND GOUT |
|
NEPHROLITHIASIS |
|
INMUNODEFICIENCIES |
|
ANEMIA |
|
DISEASES OF THE PERIPHERAL AND CENTRAL NERVOUS SYSTEMS |
MYOPATHIES |
|
Some enzyme defects of carbohydrate metabolism (glycogen storage diseases) have been associated with hyperuricemia and gout ( Fig. 3-2 ). Type I glycogenosis, due to glucose-6-phosphate deficiency, compromises glucose dephosphorylation and may increase serum urate concentrations via a dual mechanism: increased lactate production, which may interfere with urinary uric acid excretion. On the other hand, hyperuricemia in type I glycogenosis may also be the result of increased hypoxanthine, xanthine, and uric acid synthesis due to enhanced ATP breakdown and increased AMP production. In glycogenosis types III, V and VII, acetyl-CoA synthesis is impaired leading to poor citric cycle efficiency with diminished ATP production. To compensate this, the adenine nucleotide cycle overproduces fumarate to fuel the activity of the Krebs cycle. The overactivity of the adenine nucleotide cycle leads to increased IMP formation from AMP that may enter the purine nucleotide degradation pathway, leading to increased uric acid synthesis (see Fig. 3-2 ).

Congenital Disorders of Purine Metabolism Causing Hyperuricemia
The synthesis of uric acid may be viewed as the result of two main processes: (1) de novo purine synthesis (i.e., the formation of purines from nonpurine compounds) leading to the nucleotides IMP, AMP, GMP, and XMP, and (2) the catabolism of these nucleotides (purine nucleotide degradation) (see Fig. 3-1 ). Two main enzyme defects have been described that markedly increase uric acid synthesis. One is located at de novo purine synthesis (PRS overactivity), and the second at the salvage pathway (HPRT deficiency) (see Fig. 3-1 ).
Phosphoribosylpyrophosphate Synthetase Superactivity
PRS superactivity (OMIM 300661) (also known as PRPP synthetase superactivity) is a rare X chromosome–linked disorder causing juvenile gout. PRS catalyzes the synthesis of phosphoribosylpyrophosphate (PRPP), which is a main substrate in the synthesis of purine, pyridine, and pyrimidine nucleotides (see Fig. 3-1 ). The disorder is characterized by hyperuricemia and gout, which are accompanied, in the most severe phenotype, by neurologic manifestations. To date, about 30 patients have been reported with this enzyme defect, which was described for the first time in 1972.
Clinical Description
PRS superactivity results in two different phenotypes. The mild phenotype is characterized by gout and uric acid urolithiasis with late-juvenile or early-adult onset. Gout arthritis is severe and is accompanied by tophi. About 75% of patients present with recurrent uric acid stones. Uric acid urolithiasis is usually the first manifestation of the disease. Blood and urine levels of uric acid are particularly increased. Acute renal failure presentation is not as common as it is in HPRT deficiency, but it has been described in a patient presenting with a uric acid renal excretion of 2400 mg/24 hr. Recurrent uric acid stone complications such as urinary tract infection, pyelonephritis, hypertension, and renal failure may develop. Heterozygous females carrying the defect do not present clinical manifestations.
The severe phenotype is usually diagnosed in early childhood or infancy due to neurologic impairment and uric acid overproduction manifestations. Sensorineural deafness, mental retardation, hypotonia, ataxia, and progressive axonal neuropathy with demyelination have been described. Heterozygous females can also present with uric acid overproduction, with uric acid lithiasis and juvenile gout, and in some cases with sensorineural deafness.
Pathophysiology
PRS catalyzes the PRPP synthesis from Mg-ATP and ribose-5-phosphate in a reaction that requires Mg 2+ and inorganic phosphate (P i ) as activators. P i enzymatic activation involves two mechanisms: P i stabilizes the enzyme loop involved in the binding of ATP, facilitating the substrate binding of Mg-ATP complex, and P i is also an allosteric competitor of the enzyme inhibitor ADP. PRS reaction is subject to inhibition in a feedback mechanism by purine nucleotides as ADP and GDP. PRPP is substrate of three enzymes of purine metabolic pathway: PRPP amidotransferase, in de novo synthesis pathway, which serves specifically as the rate-limiting reaction for the purine synthesis, and HPRT and APRT in the salvage pathway. In addition to de novo synthesis of purine, PRPP is used in the pyrimidine and pyridine nucleotide synthesis. PRPP is cofactor for uridine monophosphate synthetase (UMPS), which converts orotic acid into UMP, the precursor of all other pyrimidine nucleotides. Finally, PRPP is utilized for pyridine nucleotide synthesis by nicotinate phosphoribosyl transferase (NAPRT) and nicotinamide phosphoribosyl transferase (NAMPT) for the nicotinamide adenine dinucleotide (NAD) and nicotinamide adenine dinucleotide phosphate (NADP) synthesis.
PRS overactivity causes an increase in intracellular PRPP, which in turn is the cause of increased purine synthesis and uric acid overproduction. However, the pathophysiology of the neurologic dysfunction remains unclear.
Although PRS activity is coded by three different genes— PRPS1, PRPS2, and PRPS3 or PRPS1L1, to date, PRS overactivity is caused exclusively by an alteration in PRPS1 (MIM 311850), which codes for PRS-I or ribose-phosphate pyrophosphokinase 1. PRPS1 is located in chromosome X, and mutations in this gene have been also associated with three syndromes in which PRS-I activity is decreased: Arts syndrome (MIM 301835), Charçot-Marie-Tooth disease-5 (MIM 311070), and X-linked nonsyndromic sensorineural deafness or DFN2 (MIM 304500). Clinical manifestations of these syndromes include sensorineural deafness, mental retardation, hypotonia, peripheral neuropathy with demyelination, ataxia, and optic atrophy.
Severe PRS superactivity phenotype is caused by point mutations in the PRPS1 gene. These mutations result in an alteration in the enzyme regulation with decreased inhibition by purine nucleotides and a higher P i affinity and activation ( Fig. 3-3 ). Mutations causing PRS-I superactivity are located by disturbing the allosteric sites, directly or by altering the homodimer interface (see Fig. 3-3 ). This fact explains the loss of feedback inhibition but may also result in a decrease protein stability. Thus, in these patients, PRS activity determined in hemolysate is usually decreased due to the instability of the mutant protein in erythrocytes. Nucleotide levels are also low in erythrocytes. These facts suggest that PRS activity could be also decreased in neuronal cells, causing neurologic symptoms that develop only in the severe phenotype. However, a mild phenotype is caused by an increased PRPS1 expression of unknown mechanism. In these patients, a higher V max is present due to a higher protein concentration and PRS activity is high in all cells tested.
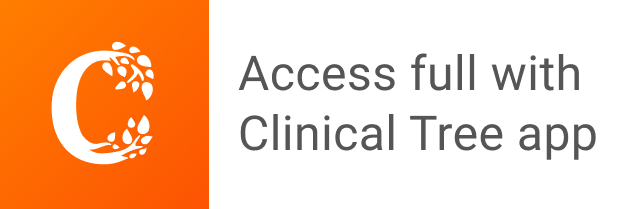