Prosthetic Gait: Biomechanical Analysis and Clinical Assessment
John T. Brinkmann MA, CPO/L, FAAOP(D)
Siobhán Strike PhD
John T. Brinkmann or an immediate family member serves as a board member, owner, officer, or committee member of the American Academy of Orthotists and the Prosthetists. Neither Dr. Strike nor any immediate family member has received anything of value from or has stock or stock options held in a commercial company or institution related directly or indirectly to the subject of this chapter.
This chapter is adapted from Queen RM, Orendurff M: Amputee gait: normal and abnormal and Brinkmann, JB, Stevens PM: Clinical considerations of observational gait analysis, in Krajbich JI, Pinzur MS, Potter BK, Stevens PM, eds: Atlas of Amputations and Limb Deficiencies: Surgical, Prosthetic, and Rehabilitation Principles, ed 4. American Academy of Orthopaedic Surgeons, 2016, pp 69-95.
ABSTRACT
An understanding of gait is essential for maximizing the mobility and function of the user of lower limb prostheses. Instrumented and observational gait analysis is valuable for guiding clinical decisions and in assessing treatment outcomes. A detailed understanding of the kinematic (motion) and kinetic (force) patterns of the hip, knee, and ankle during walking in individuals without limb absence will aid the clinician in restoring a competent, efficient, and adaptable gait pattern in prosthesis users and improve functional performance in real-world locomotive tasks. Gait deviations in prosthesis users are complex and dynamic and influenced by the level of limb loss and the prosthetic components chosen by the clinician. Understanding how patient factors (eg, strength and range of motion) and prosthetic factors (eg, fit and alignment) influence gait is essential for effective prosthetic care.
Keywords:
gait analysis; gait deviations; joint kinetics and kinematics; observational gait assessment; prosthetic alignment
Introduction
The overall goal of rehabilitation after lower limb amputation is to support a level of function commensurate with the individual’s functional capabilities before amputation. Walking is a common activity of daily living and regaining walking ability is a high priority for most individuals following an amputation.1,2 Successful walking involves transporting the body safely and efficiently from one place to another. To achieve this, each limb must support the body in turn and advance forward while transitioning weight bearing from one limb to the other. During prosthetic gait this is achieved by matching, as closely as possible, the patient’s preamputation kinetic and kinematic gait parameters. However, practitioners should expect that structural asymmetries caused by amputation will result in kinetic and kinematic asymmetries even after successful rehabilitation.3,4
Prosthetic gait that deviates excessively from normal parameters can have detrimental physical and functional effects, including inappropriate distribution of forces on the residual limb, increased loading of the contralateral limb, and increased energy expenditure.5,6,7 Long-term altered biomechanics can contribute to the development of orthopaedic problems, including osteoarthritis.8,9 Gait deviations should be minimized as much as possible to mitigate these deleterious effects.
Gait deviations are observed and addressed by applying established clinical principles and professional judgment to arrive at the most appropriate gait pattern for each individual. Instrumented gait analysis (IGA) can be used to identify, quantify, and understand the effect of lower limb absence and can facilitate appropriate prescription and clinical decision-making for patients. This chapter explores the mechanics of walking, determined through IGA, to explain the changes that occur when using a prosthesis. The relationship between these biomechanical principles, important clinical phenomena determined through observational gait assessment, and prosthetic implications are discussed throughout the chapter.
Biomechanical Goals of Human Locomotion
To be able to progress stably and efficiently, the lower limbs act as a system of rotating elements coupled in series. Each segment stores and releases energy through passive absorption of strain energy or through active muscle activation. The linked segments, known as the kinematic chain, enhance the range of motion and overall load-bearing capacity of the body. This results in a multifaceted, complex system. Prosthetic replacement of an absent limb alters
this highly coordinated system and can alter an individual’s ability to move effectively.
this highly coordinated system and can alter an individual’s ability to move effectively.
Progression
The ultimate goal of walking is to be able to move the body forward using the intact body segments and the prosthesis for support and to enable propulsion. The body’s center of mass (CoM) follows a wave-like sinusoidal motion. The body descends forward from its maximum height during single support to the low point during double support, and then must be lifted forward and upward again to rise from double support to the subsequent single-limb support. An input of energy from both limbs through concentric muscle contractions is required to enable the step-to-step transition, with the trail leg pushing and the lead limb pulling the body onto the lead limb. Eccentric muscle contractions act to control the body as it falls sequentially from one limb to the other. By understanding the primary mechanisms by which the muscles and joints act to enable progression, the requirements of the prosthesis and how its alignment can influence progression can be understood. Adequate step length, achieved through foot clearance in swing, is required for effective progression. The length and frequency of each step determine walking speed, which is a key measure of rehabilitation and functional capability. The speed of walking reduces with higher levels of amputation and less responsive prostheses.
Stability
Progression can only be accomplished if the limbs can support the body in single support and can safely transfer load from one limb to the other. To be able to walk, the stance foot must be stable on the floor and the swinging limb must be able to clear obstacles. Stability is challenged during walking because the body is top heavy and the body segments are constantly moving, so dynamic stability is required for effective walking. The reduced proprioception and altered walking pattern of people with lower limb absence has been associated with poorer balance and responsiveness to perturbations10 and increased fall risk.11 This is heightened by inability to compensate and adapt to changing walking surfaces.12 A prosthesis must be sufficiently stiff to enable stable contact with the floor and sufficiently compliant to enable progression. In an attempt to reduce the risk of falling, prosthesis users evoke a larger margin of stability compared with people with no disability.13
A more recent interpretation of stability also includes analyzing the variability of the repeating signal, which can indicate fluctuations between gait cycles.14,15 Slight differences between gait cycles are normal as one stride rarely exactly matches the next stride because of the highly complex and coordinated link-segment human body. Some variability is desirable as it allows for adaptations to the environment. However, too much variability between stride cycles has been associated with inefficiency and falling.11,16 Furthermore, if there is little variability between movement cycles (every stride is similar to the ones before and after) the walker is unable to adapt to different stimuli or to the changes in the environment, such as a nudge or changing surface, and may lose confidence when walking in the community. The effect of limb absence, prosthetic componentry, and alignment on gait variability is not well understood. Further research is required to determine the appropriate healthy thresholds of variability which is neither too great (leading to inefficiency and poor balance control) nor too low (leading to reduced ability to adapt to the environment).14
Efficiency
To be sustainable, walking must not be too tiring. In normal walking, the interplay between kinetic and potential energy through the gait cycle, coupled with synergistic muscle contractions across joints, along with making use of muscle stretch during an eccentric contraction before a concentric contraction (the stretch-shortening cycle), reduces the metabolic cost required to progress the body. The energy cost of walking increases with more proximal levels of limb absence as a consequence of the missing anatomy, and can be influenced by prosthetic technology. Habitual self-selected walking speed can be used as an indicator of efficiency as energy expenditure is normally lowest at this speed.
When walking with a prosthesis, impaired control, poor proprioception, muscle absence and weakness, and pain all may challenge the body’s ability to achieve an efficient and symmetrical step-over-step walking pattern. The prosthesis should make use of all the force and energy resources, both internal and external to the body, to enable progression which is energy efficient but not at the cost of maintaining a stable base of support. The level of amputation, mechanics and alignment of the prosthesis, and the type and fit of the socket will affect walking ability and efficiency. For example, the gait deviations observed during transfemoral prosthetic gait are more common and more noticeable than those associated with transtibial prosthetic gait. The relatively bony nature of the transtibial limb generally facilitates control of the prosthesis, and the redundant soft tissues of the transfemoral limb often present a challenge in achieving consistent prosthetic control. In addition, the shortened anatomic lever arm of the more proximal residual limb, the increased lever arm of the prosthesis, and additional loss of muscular control of the residual limb further reduce a patient’s ability to control their gait pattern while wearing a transfemoral prosthesis. This is evident throughout the gait cycle and across all planes, but in particular in the coronal plane instabilities common at the transfemoral level, and in the challenges in maintaining sagittal plane knee stability during standing and ambulation.
Phases of the Gait Cycle
Walking is a cyclic activity-the movement is repeated over and over and ideally should be symmetrical between the two limbs. The gait cycle is used to give a framework for analysis. It is now well accepted that the gait cycle starts and ends with initial contact of one foot with the floor. A stride is defined from
initial contact of one foot to the next initial contact of that foot, whereas a step is initial contact of one foot to the initial contact of the other foot. Step is the term often used clinically to describe the portion of this cycle when the limb is in the swing phase. For more clarity, the swing and stance phases of a step should be described explicitly when referring to a step.
initial contact of one foot to the next initial contact of that foot, whereas a step is initial contact of one foot to the initial contact of the other foot. Step is the term often used clinically to describe the portion of this cycle when the limb is in the swing phase. For more clarity, the swing and stance phases of a step should be described explicitly when referring to a step.
The gait cycle is broadly divided into two phases: stance and swing. The stance phase is defined by the foot being in contact with the ground and swing is defined by the foot moving through the air. The stance phase comprises approximately 60% of the gait cycle and the swing phase about 40%. Single support is defined as only one foot is in contact with the ground while the contralateral limb is in swing. There are two periods of double support, when both limbs are in stance, each comprising about 10% of the gait cycle. (Double support distinguishes walking from running, which has no double support and involves a period of flight when neither foot is in contact with the support surface.) The first double support phase is frequently known as the loading phase whereas the second double support phase is frequently called the propulsion phase (Figure 1).
Different subphasing systems are used and the two most common are Perry’s five phases of stance and three of swing17 and Kirtley’s three phases of stance and two phases of swing18 (Figure 1). Kirtley’s phases are becoming more popular, make the dynamic walking theory (outlined in the next section) easier to conceptualize, and allow a sensible interpretation of the coordination of both limbs as they achieve their tasks simultaneously. Loading of the ipsilateral limb, which is in front of the CoM, causes it to decelerate. At the same time, the contralateral limb is in propulsion, accelerating the CoM. This interaction has been established as important to achieving progression.
Theoretical Models of Gait
Different models have been used to analyze how individuals walk, and the simplest models consider the movement of the CoM. Horizontal and vertical velocity are not constant. During loading, the lead limb is in front of the CoM and acts to decelerate it and to ensure the forward motion of the trunk. The muscles of the lead limb must contract to accelerate the body forward and upward. This process costs energy. After World War II, one of the earliest quantitative gait analyses of normal and prosthetic gait described six determinants of gait19 to explain these energy changes. This analysis detailed the individual and integrated requirements of joint rotations and suggested that the limbs act to minimize the vertical movement of the CoM. However, although this model is conceptually useful, it is not generally used because it is limited in application—the energy cost of walking is not fully explained using this model because the CoM oscillates more than the model predicts.
Cavagna et al20 developed an alternative model, which considered the body as an inverted pendulum. The CoM moves in an arc-like trajectory while sitting on top of a rigid pendulum (the leg) during stance, using gravitational potential energy for forward progression. Although this is an informative model, the energy cost of walking is much greater than the model suggests. This indicates the limitations of considering the limbs as nonjointed rods and highlights the importance of flexion and extension at the joints to achieve efficient progression.
The dynamic walking model has recently gained widespread acceptance21 (Figure 2). This model highlights the importance of the step-to-step transition
between each single support. The transition emphasizes the need to redirect the CoM velocity when moving from one leg to the next. This model makes a convincing argument that the push from the trailing limb in late stance balances the braking from the leading limb in early stance to allow a smooth transition from one limb to the next. This theory provides a useful framework to understand why the limbs act as they do and why particular compensatory mechanisms are adopted or pathologies result. For example, the theory explains why the energy cost of walking is higher for people using a prosthesis as the trailing prosthetic limb cannot push the leading, contralateral intact limb into its stance. The intact leading limb experiences higher forces and increased energy contributions to enable the step-to-step transition. Because the prosthesis does not effectively accelerate the body over the intact side, the intact side has to work harder and experiences greater forces to compensate.22
between each single support. The transition emphasizes the need to redirect the CoM velocity when moving from one leg to the next. This model makes a convincing argument that the push from the trailing limb in late stance balances the braking from the leading limb in early stance to allow a smooth transition from one limb to the next. This theory provides a useful framework to understand why the limbs act as they do and why particular compensatory mechanisms are adopted or pathologies result. For example, the theory explains why the energy cost of walking is higher for people using a prosthesis as the trailing prosthetic limb cannot push the leading, contralateral intact limb into its stance. The intact leading limb experiences higher forces and increased energy contributions to enable the step-to-step transition. Because the prosthesis does not effectively accelerate the body over the intact side, the intact side has to work harder and experiences greater forces to compensate.22
The acceleration of the center of mass is reflected in the ground reaction force (GRF) which has direction and magnitude, represented by a vector (GRV). The body’s momentum is related to the impulse (force × time) generated under the feet. The vertical acceleration of the CoM can be interpreted through the vertical GRF (vGRF)22,23 (Figure 3). The higher magnitude of the forces on the intact limb24 (Figure 3, dotted lines) may be a reason for loading-related comorbidities such as joint pain and osteoarthritis at joints of that limb.25 This illustrates the limited capacity of the prosthesis to replace the active contractions by the muscles to accelerate the body when walking, evidenced by the lower magnitude of vGRF (Figure 3, solid lines). A value of 10 N/kg equates to almost one body weight (BW). In walking, magnitudes of a little over 10 N/kg are typical, and these will increase with faster walking and reduce with slower walking. Magnitudes of approximately 15 to 25 N/kg are experienced during jogging and running, while values of over 30 N/kg may be experienced when landing from a jump.
Instrumented Gait Analysis
Walking is the most common activity of daily living and also one of the most researched human movements, allowing a relatively good understanding of how the anatomy and mechanics work to produce movement. Using quantitative IGA (objective measurement of specific variables) has enhanced understanding of how missing anatomic segments can be more effectively replaced. Gait analysis allows identification of the causes of altered joint actions and how different prosthetic designs affect them. More sophisticated prostheses,24 better fitting sockets,26 and greater attention to appropriate alignment27 have resulted in improved walking patterns for prosthesis users.
Four main types of data can be recorded, often simultaneously, depending on the level of sophistication of the IGA system: temporospatial, kinematics, kinetics, and electromyography. Of these, temporospatial variables are the least complicated and expensive to collect and analyze but are not able to identify the cause of the deviation. Kinematic variables relate to those that can be seen-linear and angular (joint) displacement, velocity, and acceleration. These require a video and/or (electro)goniometer to record the motion. Careful placement of the equipment is required to acquire valid and reliable data. Kinetic data require the measurement of forces. Quantifying kinetic data requires expensive equipment and specialist training for interpretation. However, this allows a better understanding of the cause and effect of the deviation. Electromyography data quantify the timing and intensity of the muscle contraction and also require specialist training and equipment. Excellent resources on how to collect and interpret gait data are available.17,18,28,29,30
Practitioners in most clinical settings do not have access to three-dimensional IGA and must rely on unassisted observation as the primary method for identifying pathologic gait patterns. Several limitations are inherent in observational assessment. First, many factors that influence gait are not directly observable. For example, the pressure of the residual limb against the socket and the corresponding joint moments caused by those pressures can only be directly observed using equipment and methods not available in most clinical settings. Second, observational gait assessment also has been demonstrated to have limited validity and reliability for gait-related events in neuromuscular and musculoskeletal pathologies.31,32 Studies focused specifically on individuals using lower limb prostheses have confirmed this limitation.33 However, because resources and expertise are limited, observational gait assessment and clinical judgment remain the primary strategies for assessing prosthetic gait clinically. With a proper understanding of the mechanics of the gait cycle, the relationship between joint moments, and the influence of prosthetic alignment, many commonly observed deviations can be resolved effectively using these clinical strategies.
Analyzing the temporal (time) and spatial (distance) characteristics of the gait cycle allows the practitioner to determine the quality of the walking performance. Along with age,34 strength,35 rehabilitation,36 engagement with physical activity,37 prosthetic components,38 time and experience using the prosthesis,39 the environment, and performing secondary tasks all have been shown to affect the temporospatial variables.
Walking speed can easily be calculated by measuring the time it takes to complete a defined distance using simply a stopwatch and measuring tape. Different lengths of walk can be used, depending on ability. Typically, walking speed is determined over 10 m. For example, if a person takes 11 s to complete 10 m, their average walking speed is 10/11 = 0.91 m.s-1. Walking speed is determined by the stride length and the stride frequency. A stride includes two steps. Step length is defined by the distance the limb moves forward in front of the supporting limb (Figure 4). The right step is defined by initial contact of the left foot to initial contact of the right foot, that is, the foot advancing during swing defines the step.
Average stride length can be quantified by dividing the distance walked by the number of strides. If a person takes seven strides to cover 10 m, their average stride length is 10/7 = 1.43 m. If the step length is symmetrical, then a step length is half the stride length.
Step length and time asymmetries are common in prosthetic gait and indicate that the remaining limbs and the prosthesis are not able to completely replace the function of the missing anatomy.40,41 Some common causes of differences associated with the prosthesis are detailed in the next section.
Analysis of joint kinematics and kinetics may be required to fully understand the cause of these asymmetries.
Analysis of joint kinematics and kinetics may be required to fully understand the cause of these asymmetries.
Prosthetic Step Length Asymmetries
Disruptions in the stance and swing ratio are common in prosthetic gait. Step length asymmetries are best observed in the sagittal plane. They can be observed as shortened steps on either side, corresponding with shortened stance periods on the contralateral side. Uneven arm swing, which is often observed during prosthetic gait, may be related to unequal step lengths caused by pain during ambulation or other factors. Clinically, the root causes for the asymmetrical step must be correctly identified in each case, and may not be completely correctable.
A short step on the nonprosthetic side is more common because the prosthetic foot and ankle cannot actively plantarflex, which limits heel rise and push-off. More mobile feet and ankles and those with dynamic energy return can mitigate this and allow a more symmetrical gait when aligned properly and of the correct stiffness. The shortened contralateral step also often results from the patient’s reluctance to maintain full body weight on the prosthesis. During propulsion phase on the prosthetic side the contralateral step is shortened by placing the foot on the ground earlier. This is particularly common early in the gait training process, and the patient should be trained to take smaller, more symmetrical steps. Less frequently, a shortened prosthetic step length may be observed. This is often the result of a flexion contracture of the contralateral knee that prevents full extension in terminal swing.
Step length asymmetries are commonly observed during transfemoral prosthetic gait. Sagittal knee stability is of paramount concern as patients transition into the support phase on their prosthetic side and begin to load their prostheses. A large prosthetic step increases the knee flexion moment on the prosthetic side during loading and can decrease knee stability. By taking a shorter prosthetic step patients can reduce the knee flexion moment and achieve greater knee control and stability. Patients may even exhibit a step-tostep gait pattern on the prosthetic side, never advancing the prosthetic foot further forward than the contralateral foot. This increases the knee extension moment on the prosthetic side, increasing stability during the support phase. Because of stability concerns, prosthesis users are often reluctant to shift their weight fully onto their prostheses and allow their body weight to transfer from the heel to the toe of the prosthetic foot throughout the stance phase. In this case, a shortened stance phase on the prosthetic side and a shortened nonprosthetic step allow the patient to offload the prosthesis earlier as they transition to the contralateral (nonprosthetic) limb.
Hip flexion contractures, which are very common at the transfemoral level, prevent the hip on the affected side from attaining sufficient extension to allow a full-length sound side step. Unfortunately, it is very common for this lack of range of motion to not be properly accommodated for in the socket alignment (insufficient initial socket flexion), and this improper alignment is a common source of step length asymmetries observed clinically. Gait training, hip range of motion, and proper alignment all are crucial to maintaining step length asymmetry at the transfemoral level.
At the transtibial level, where knee stability is often less of a concern, patient confidence in the support provided by their sound limb during the stance phase results in them taking a longer step with their prosthetic limb and a relatively shorter step with the nonprosthetic limb. This deviation is especially common in newer amputees and can become habitual if not identified and corrected early in gait training. Improvements may be seen with time, training, and improved confidence in the prosthesis. However, poor habits developed during initial gait training can be very difficult to correct later. During gait training, more emphasis should be placed on step asymmetry achieved by a relatively shorter step on both sides, and developing the habit of loading the prosthetic foot in mid and terminal stance, rather than on a large initial step on the prosthetic side. Instructing the patient to take their first step with the nonprosthetic limb can help them develop confidence in the support provided by the prosthesis during terminal stance.
Kinematics and Kinetics of Gait
In the 1980s to 1990s three seminal research teams codified the gait cycle, highlighting the variables that are commonly used to complete a clinical gait analysis (joint angles, moments, and powers) and their interpretation.17,28,42 Kinematic variables typically describe the joint angles (Figure 5, A through C) through the gait cycle, whereas kinetic variables relate to the joint moments (Figure 5, D through F) and powers (Figure 5, G through I). IGA systems which involve motion capture and force plates allow quantification of the kinematics and kinetics of gait. These variables are interpreted in the context of the requirements of each subphase of the gait cycle (Figure 1) and the mechanisms that are required to meet these demands.
Internal joint moments indicate the net force of the muscles that act to cause rotation at the joint. These muscle moments resist the inertial forces/moments produced by the external GRV. If the joint velocity and moment are in the same direction, a concentric contraction is occurring at the joint. Positive power indicates this type of contraction. When muscles contract concentrically (shortening) they act as accelerators and energy generators. If the joint velocity and moment are in opposite directions, an eccentric contraction is occurring. When muscles contract eccentrically (lengthening) they act as decelerators and energy absorbers. A negative power indicates an eccentric contraction. For example, in single support, the ankle is dorsiflexing while the plantarflexors are contracting. As the joint velocity and moment are in opposite directions, a negative power burst indicates this eccentric contraction by the plantarflexors to control the progress of the leg over the foot during the second ankle rocker, known as A1S. However, in late single support
and double support propulsion, the ankle is plantarflexing with an internal plantarflexor moment. The velocity and moment are in the same direction and a positive power burst indicates this concentric contraction to lift the limb into swing and shift the weight onto the contralateral limb, known as A2S.
and double support propulsion, the ankle is plantarflexing with an internal plantarflexor moment. The velocity and moment are in the same direction and a positive power burst indicates this concentric contraction to lift the limb into swing and shift the weight onto the contralateral limb, known as A2S.
The specific power bursts in the sagittal plane (Figure 5, G through I: A1S, A2S, K1-4S, H1-3S) and in the frontal plane (H1-2F) were detailed by Winter28 and Gage42 and are referred to in the following sections. Their research highlighted the key variables associated with effective walking gait, though the relative contribution of these variables is still controversial, particularly with respect to the relative importance of the ankle push-off power in the propulsion phase. Sagittal plane angles, moments, and powers are often the focus of walking research because the movement is greatest in this plane, to enable forward progression.
The effect of reduced motion and function of the prosthetic ankle can be seen in the reduced angles, moments, and powers at the knee and hip.43 The ankle, knee, and hip joint angles indicate reduced range of motion on the prosthetic side throughout the gait cycle (Figure 5, A through C). Joint moments indicate reduced moments on the prosthetic side, particularly at the ankle (Figure 5, D) and knee (Figure 5, E) which has been associated with reduced strength because of long-term disuse.35 Joint powers indicate reduced powers on the prosthetic side, particularly at the ankle (Figure 5, G), indicating the inability of the prosthesis to actively alter the joint orientation, which limits the user’s ability to walk at different speeds.44
The Loading Phase
During double support loading, the limb must be able to accept weight and transition from the trailing leg (Table 1). The limb usually contacts the ground with the heel, with the ankle slightly dorsiflexed (Figure 5, A: 0° to 10°), the knee slightly flexed (Figure 5, B: 5° to 15°), and the hip flexed (Figure 5, C: 30° to 40°). The GRV is behind the ankle and knee and in front of the hip, tending to cause the ankle to plantarflex and the knee and hip to flex. As the foot rolls to foot flat, the ankle slightly plantarflexes, which is controlled by the dorsiflexors contracting eccentrically (creating an internal dorsiflexion moment) to control the lowering of the foot to the floor (Figure 5, D). Plantar flexion of the foot during this phase has been described by Perry17 as the first rocker.
Knee flexion is controlled by eccentric contraction of the extensors (creating an internal knee extension moment) (Figure 5, B, E, and H). This serves as a power-absorbing mechanism (K1S) that facilitates the smooth foot-to-foot transition (Figure 5, H). The hip extends as a result of a concentric contraction by the hip extensors (H1S), actively pulling the body up toward single support (Figure 5, G and I).
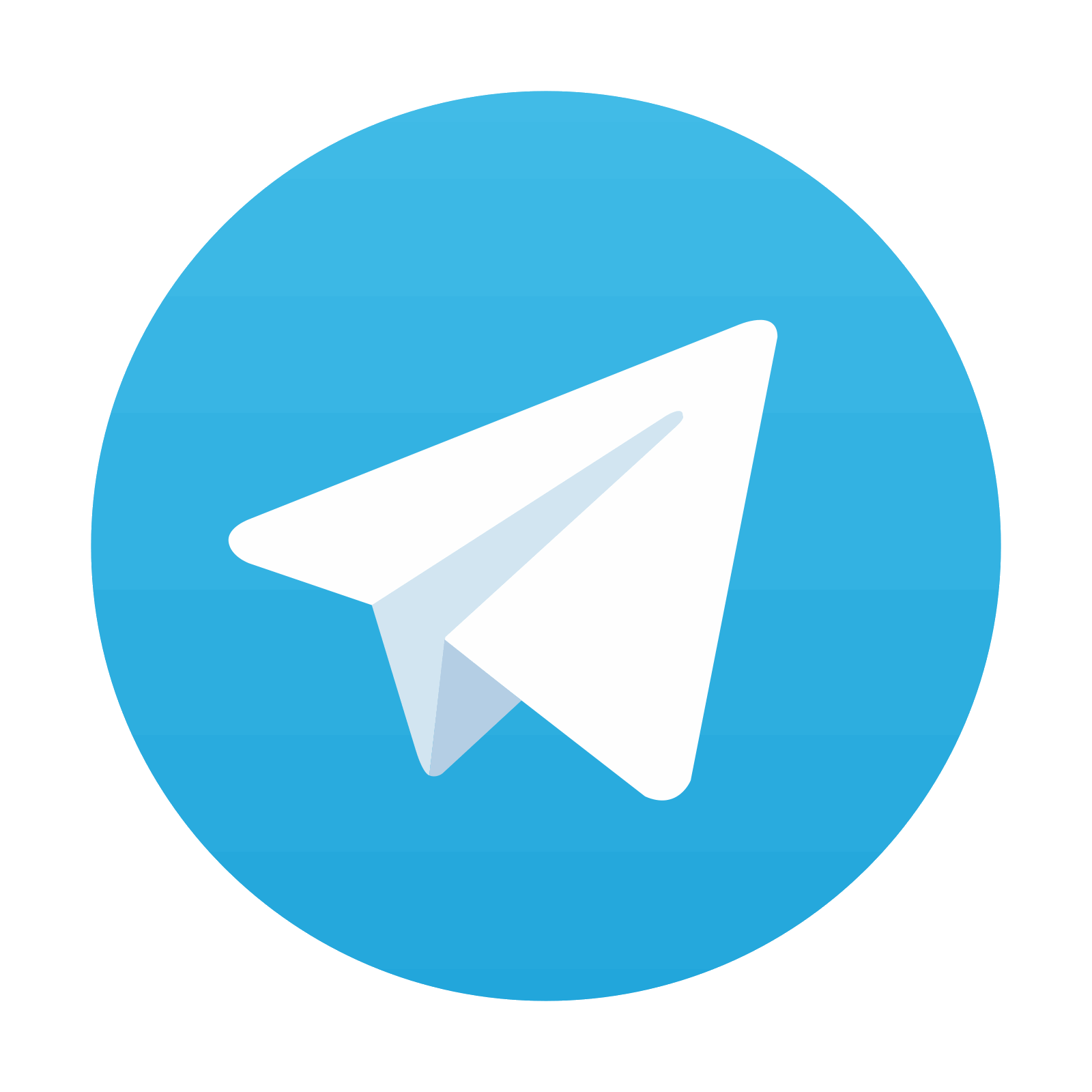
Stay updated, free articles. Join our Telegram channel
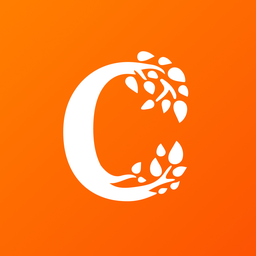
Full access? Get Clinical Tree
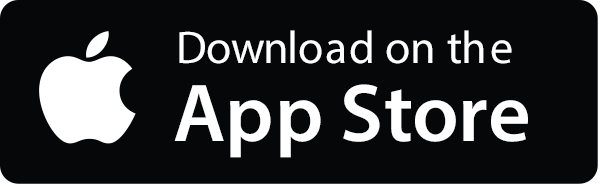
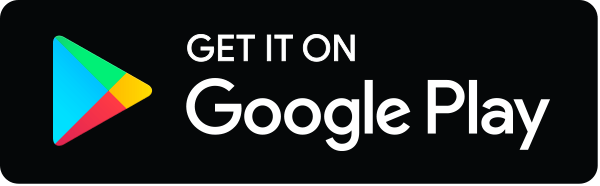