Pearls
- •
Hemodynamic monitoring refers to measurement of the functional characteristics of the heart and circulatory system that affect the perfusion of tissues with oxygenated blood.
- •
Hemodynamic monitoring can be performed invasively or noninvasively and can be used for diagnosis, surveillance, or titration of therapy.
- •
The central venous waveform is composed of three waves ( a , c , and v ) and two wave descents ( x and y ).
- •
The arterial waveform has three components: rapid upstroke, dicrotic notch, and runoff.
- •
Pulse pressure variation has excellent specificity as an indicator of fluid responsiveness in many critically ill patients.
- •
Cardiac output can be calculated using the Fick method or measured directly via thermodilution.
- •
A pulmonary artery catheter can be used to measure cardiac output and indices of oxygen delivery and extraction.
Role of invasive hemodynamic monitoring
Since William Harvey’s observation in the early 1600s that the heart pumps blood in a continuous circuit, the function of the circulatory system has been the subject of intense scrutiny. Hemodynamic monitoring refers to measurement of the functional characteristics of the heart and circulatory system that affect the perfusion of tissues with oxygenated blood in order to maintain homeostasis and to remove byproducts of metabolism. Several different types of invasive hemodynamic monitoring can be used concurrently to guide management. The goal of hemodynamic monitoring is to provide accurate diagnoses and to guide additional interventions to deliver improved care to the critically ill patient.
In his 1733 report “Statical essays: containing haemastaticks; or, an account of some hydraulick and hydrostatical experiments made on the blood and blood-vessels of animals,” Hales described early experiments in horses in which he used tubular devices inserted directly into arteries to measure intravascular pressures. Fig. 26.1 depicts Hales and an assistant in the process of these early experiments. This figure also illustrates a simple method for inferring arterial versus venous placement of a vascular catheter, which can also give a quick bedside estimate of central venous pressure.

Frequently in the pediatric intensive care unit (PICU), noninvasive assessments of hemodynamics are supplemented by invasive hemodynamic measures that require entrance into the intravascular space. Such invasive hemodynamic measurements include placement of central venous catheters (CVCs), arterial catheters, and pulmonary artery catheters (PACs). Invasive hemodynamic monitoring can provide the skilled intensivist with a plethora of valuable information but should still be integrated with all patient data rather than viewed in isolation. Successful use of invasive hemodynamic measurements necessitates skills to obtain these measures safely with attention to the risks imposed on the patient. As with any technology, the use of invasive hemodynamic monitoring is in evolution, and it is incumbent on the clinician to be familiar with developments as they arise.
This chapter aims to be a practical guide to the use of hemodynamic monitoring in the PICU. It reviews general principles of measurement and discusses the three main types of invasive hemodynamic monitoring: CVC, arterial catheter, and PAC. It addresses the indications and controversies, interpretation of waveforms, and potential complications and also reviews cardiac output (CO) monitoring and calculation of oxygen consumption and delivery. New techniques coupling invasive monitoring with noninvasive devices are also discussed. The specific techniques for gaining access to make these measurements are detailed in Chapter 14 , which covers invasive procedures.
Indications for invasive hemodynamic measurements
The three main indications for invasive hemodynamic monitoring are diagnosis, surveillance, and titration of therapy. Diagnosis may include the differentiation of septic shock (through assessment of factors such as diminished right heart filling pressures or preload and decreased systemic vascular resistance) from cardiogenic shock (characterized by elevated left heart pressures and afterload). Surveillance implies observation over time. The purpose of surveillance may be to assess the stability of a patient at risk for adverse changes or to determine the response to therapy. Invasive measurements performed for diagnostic purposes often are continued for surveillance. Titration of therapy is often based on information gleaned from invasive measurements.
Principles of measurement
Intensive care clinicians rely on a wide variety of measurement systems to assess patient clinical status and response to therapy. However, not all clinicians have a good understanding of how physiologic variables are measured and, consequently, may not be able to troubleshoot monitoring systems or recognize when information obtained is inaccurate. A detailed discussion of monitoring is beyond the scope of this chapter, but a basic understanding of the principles of measurement is helpful in deciding which measurements to trust and how to assess a monitoring system for accuracy. Detailed descriptions of monitoring systems are provided elsewhere.
Signal analysis
Measurements generally are made directly by comparison with known standards or indirectly by use of a calibration system. Determination of length or weight usually is made by direct comparison with a standard ruler or standard mass. Most invasive measurements in the ICU are made indirectly, thereby requiring use of a calibration system. Thus, understanding the basis for calibration of a system is important to determine the validity of the measurement.
Measurement systems detect and transform signals so that they can be presented in an interpretable way to the user. Signals can be characterized as static or dynamic. Slowly changing signals, such as body temperature, can be thought of as static. Hemodynamic measurements change from moment to moment and thus are dynamic. Physiologic signals may be periodic; for example, arterial pressure is periodic because it varies with the cardiac cycle.
Complex periodic signals, such as an arterial pressure waveform, can be described mathematically as the sum of a series of simpler waveforms, called a Fourier series . Alternatively, the arterial tracing can be thought of as a sum of simpler waveforms, sine waves, and cosine waves. Fig. 26.2 depicts an arterial pressure waveform as the sum of the first six terms in the Fourier series. The sum of the first six terms in the series forms a waveform similar to the original tracing. Adding terms from the Fourier series, or higher harmonics, results in an increasingly better representation of the actual waveform. In general, to reproduce a pressure tracing without loss of significant characteristics for clinical use, the measurement system must have an accurate frequency response to approximately 10 times the fundamental frequency (first 10 harmonics).

The sampling rate of a measurement system determines how often a physiologic value is measured. For body temperature, sampling every few minutes might be sufficient, but for arterial pressure measurement, a higher rate is necessary. This principle may seem obvious; however, as an example of the importance of sampling rate, consider the number of points needed to define a circle. If three equidistant points are placed on a circle, a triangle is described, not a circle. Similarly, four points describe a square. If the number of points (sampling rate) is increased, the circle is described more completely. For a sine wave, the minimum frequency of sampling needed to preserve the waveform is twice the frequency. This mathematical minimum is known as the Nyquist frequency . For complex waveforms, such as arterial pressure tracings, the sampling rate must be at least twice the highest frequency component in the waveform.
Measurement systems
Hemodynamic monitoring in the clinical setting usually uses a fluid-coupled system in which changes in pressure are transmitted via a column of (ideally incompressible) fluid in an (ideally incompressible) tube to a mechanical transducer. The mechanical transducer, usually a displaceable screen diaphragm, converts a change in pressure to an electrical signal, which can be processed and displayed. In laboratory settings, vascular pressures can be measured by a transducer at the point of interest rather than remotely, as in the clinical setting. Measuring pressure at the point of interest—directly in the aorta, for example—decreases loss of signal integrity because of the measurement system. Most clinical pressure measuring systems have sufficient fidelity for clinical purposes. However, compliance, resistance, or impedance in the pressure tubing can result in damping or alteration of the recorded signal. Care should be taken to ensure that the length of the tubing is not overly long, as this can lead to resonance of the physiologic signal. Similarly, the narrower the tubing used in the system, the more resonant it will be. The presence of bubbles in the fluid can further damp the recorded signal.
Errors in measurement
The ideal measurement system determines the actual or “true” value for the measured variable. However, determination of a true value may be difficult. Every measurement system is subject to various errors. Errors in measurement can be classified as either systematic or random. Systematic errors occur in a predictable manner and are reproduced with repeated measures. Bias in a measurement system—for example, a baseline offset—results in a systematic error. Random errors are unpredictable and do not recur predictably with repeated measures.
Accuracy of a measurement is defined by the difference between the measured and true values, divided by the true value. Precision is defined by the reproducibility of the measurement; thus, a more precise system yields more similar values for repeated measures under the same conditions than does a less precise system. Imprecision can be thought of as a representation of random errors, whereas bias can be thought of as a representation of systematic errors.
Calibration
Many measurement systems are linear, that is, based on an assumption that the relationship between the inputs and outputs from a measurement device can be fitted to a straight line. This assumption allows a system to be calibrated under two conditions, with the rest of the values falling on the line defined by those two points. Actual nonlinearity of the system adversely affects the measurements.
Calibration is a process in which the reading, or output of a device, is adjusted to match a known input value. For example, an electronic pressure transducer may be calibrated against a mercury manometer. If the input to the device is zero, the output should be adjusted so that the reading also is set to zero. This “zeroing” reduces any baseline offset, thus reducing systematic errors in subsequent readings. The system then is calibrated to a nonzero value, for example, 100 mm Hg pressure, and the system gain is adjusted to read this value as well.
Frequency response
The ability of a measurement system to accurately measure an oscillating signal, such as arterial blood pressure, is dependent on the system’s frequency response. The system can either overestimate or underestimate the true amplitude of a signal. If the system is overdamped, the value reported underestimates the amplitude, and waveform characteristics may be lost. Resonance in the system may result in overestimation of the amplitude. Measurement of arterial systolic pressure—the amplitude of the arterial waveform—may be inaccurate because of overdamping, and important waveform characteristics may be lost if the frequency response of the measurement system is poor.
Impedance
Impedance is the ratio of the change in blood flow along a vessel to the change in the pressure in the vessel. Impedance has both resistive and reactive components. In a pulsatile system such as the cardiovascular system, resistance alone does not fully describe the impediment or impedance to forward flow of blood. The caliber, length, and arrangement of the blood vessels and the mechanical properties of the blood (such as its rheology and viscosity) determine resistance in the blood vessels. Reactance includes compliance of the vessels and inertia of the blood and thus is a dynamic component of impedance. This is important because the pulsatile nature of the cardiovascular system is dynamic.
When blood is propelled through a vessel at a branch point, a reflected pressure wave back toward the heart increases the impedance of the system. The major sites of wave reflection from vessel branching are from vessels approximately 1 mm in diameter. Thus, these small vessels contribute significantly to overall impedance. Fig. 26.3 shows the relationships between pressure and flow velocity with distance along the length of the aorta. Because blood pressure increases with distance from the heart and flow velocity decreases with distance, the impedance increases toward the peripheral vasculature. Hemodynamic measuring systems are essentially physical extensions of the vascular system; thus, the configuration and characteristics of the tubing and transducer system can alter the overall effect of impedance.

Invasive techniques
Central venous catheters
Indications
Indications for CVC placement in pediatric patients include assessment of central venous pressure (CVP), monitoring of large fluid shifts between the intravascular and extravascular spaces, infusion of vasoactive substances, monitoring central venous oxygen saturation, and infusion of hyperosmolar fluids and/or irritants.
Interpretation of waveforms
CVP is ideally a measure of right atrial pressure, although it may be measured in the inferior or superior vena cava (SVC). It is a measure of preload—the force or load on the right ventricle during relaxation or filling. CVP is measured at the end of diastole, just prior to ejection. Final filling of the right ventricle occurs at the end of atrial contraction. When the tricuspid valve is open during diastole, the right atrium and right ventricle form a continuous column; therefore, right atrial pressure reflects right ventricular end-diastolic pressure. CVP is used to measure filling pressure or preload and is an indicator of volume status; however, it is influenced by a variety of physiologic phenomena. It is commonly used in patients with hypovolemic or septic shock in whom volume resuscitation is desirable prior to institution of vasopressor therapy. In patients with decreased right ventricular function or pulmonary hypertension, an increased CVP well beyond normal limits may be observed and further fluid resuscitation may contribute to the development of congestive heart failure. Increases in positive end-expiratory pressure can decrease preload despite an increased CVP. Finally, increases in extrathoracic pressure, such as that caused by increased abdominal distension, can increase CVP.
It is important to keep in mind that CVP as an absolute number does not necessarily indicate fluid status due to its many physiologic determinants (stressed venous volume, venous compliance, venous resistance, right heart function, and sympathetic tone, among others). At the extremes (i.e., <6–8 or >12–15 mm Hg), CVP has satisfactory performance as an indicator of fluid responsiveness. Additionally, the change in CVP (or its staying the same) during therapeutic interventions or diagnostic maneuvers has been shown to perform better than the absolute number. As with most widely available measurements, the widespread availability of CVP at the bedside has many limitations but, when integrated into other data, frequently provides important information.
The CVP waveform is divided into three components: a , c , and v waves ( Fig. 26.4 ). Each component can be correlated with a specific portion of the electrocardiogram (ECG) tracing. The a wave occurs with atrial contraction and is seen after the P wave of the electrocardiogram during the PR interval. Thus, the mean value of the a wave approximates right ventricular end-diastolic pressure. Cannon a waves ( Fig. 26.5 ), which are enlarged a waves seen when the right atrium is ejecting against a closed tricuspid valve, may be seen when atrioventricular discordance occurs (i.e., during junctional ectopic tachycardia, ventricular tachycardia, or heart block). The c wave occurs in early systole with closure of the tricuspid valve and is seen at the end of the QRS complex in the RST junction. The v wave occurs during filling of the right atrium in late systole before opening of the tricuspid valve and is seen between the T and P waves of the ECG. The v wave is increased in the setting of tricuspid regurgitation. The x descent is the decrease in pressure after the a wave, reflecting atrial relaxation. The y descent is the decrease in pressure that occurs after the v wave as the tricuspid valve opens and passive filling of the right ventricle occurs.


Mixed venous oxygen saturation
Mixed venous oxygen saturation (Sv o 2 ) can be measured intermittently by blood sampling from a CVC or continuously by using a specially designed CVC. Such catheters typically have two to three lumens and have the same capabilities of standard CVCs, with the additional potential for spectrophotometric monitoring. The Sv o 2 catheters use reflection spectrophotometry and are able to read hemoglobin oxygen saturation continuously. The reflected light is dependent on the oxygenated and deoxygenated hemoglobin concentration in the circulating blood.
Sv o 2 measurement may be used to inform the practitioner of the relationship between oxygen delivery and consumption and is often used as a surrogate for cardiac index (CI). Rivers et al. showed that when continuous Sv o 2 monitoring was used to guide resuscitation and hemodynamic support in patients with severe sepsis and septic shock, survival rates improved. Guidelines set forth by the American College of Critical Care Medicine/Pediatric Advanced Life Support have recommended goal-directed therapy with a target Sv o 2 of 70% or more in children and adolescents who are in septic shock. Rivers’ findings have subsequently not been replicated, and continuous monitoring of Sv o 2 has not been shown to improve patient outcomes in large well-conducted studies. However, since Rivers’ initial report on goal-directed therapy, there have been sustained improvements in sepsis mortality that have coincided with an increase in awareness of the importance of monitoring end-organ perfusion. This has led to the development of many new noninvasive techniques for perfusion monitoring.
When placing CVCs for monitoring, the catheter should be placed such that the tip taking measurements is at the cavoatrial junction. Sv o 2 measurements obtained from the inferior vena cava exhibit greater variability because of fluctuations in splanchnic oxygen utilization and thus are less reliable. Sv o 2 measurements from the right atrium contain coronary sinus blood and are more desaturated because of the high oxygen extraction rate of the myocardium. Studies in critically ill children have evaluated Sv o 2 measurements obtained in the pulmonary artery and SVC. Concordance analysis showed appropriate agreement in the measurements between these two sampling sites. This finding has clinical importance because the use of PACs has declined and CVC use has been increasing. ,
Arterial pressure catheters
Indications
The transition to direct monitoring of arterial blood pressure dates back to the mid-1950s when two separate studies compared invasive arterial measurements and noninvasive or cuff measurements in healthy adults. , Van Bergen et al. noted a frequent difference between direct and indirect measurements, with indirect measurements increasingly lower than direct measurements as the systemic blood pressure increased. The greatest disparity was found in young hypertensive patients. Similarly, Cohn and Luria observed that invasive arterial pressures were significantly greater than cuff pressures and emphasized the importance of direct measurements of systemic arterial pressure when caring for patients with hypotension and shock. Continuous direct monitoring of arterial blood pressure should be considered when treating patients who require more than minimal vasopressor therapy.
Indications for arterial catheterization include continuous monitoring of systemic arterial blood pressure, frequent blood sampling, and withdrawal of blood during exchange transfusions. The procedural information regarding site selection and procedural techniques for placing arterial lines is covered elsewhere in this text.
Interpretation of waveforms
The arterial waveform has three main components: (1) a rapid upstroke and downslope that correlates with systolic ejection, (2) a dicrotic notch that correlates with closure of the aortic valve, and (3) a smooth runoff that correlates with diastole. The dicrotic notch or incisura is decreased in situations of hyperdynamic CO in which left ventricular output and stroke volume (SV) are increased, pulse pressure is widened, and diastolic blood pressure (DBP) is increased (e.g., surgical systemic-to-pulmonary shunts, patent ductus arteriosus, aortic regurgitation, anemia, fever, sepsis, hypovolemia, exercise). Conversely, cardiac tamponade and severe aortic stenosis can narrow the pulse pressure and are associated with a deflection (anacrotic notch) on the ascending limb of the waveform.
Systolic pressures measured in the periphery typically are greater than those measured more centrally because of pulse amplification of pressure waves reflected back from arterial branch points , (see Fig. 26.3 ). More peripheral sites, such as the radial artery, have greater systolic blood pressure (SBP) and lower DBP than more central sites and thus taller and narrower waveforms with greater pulse pressures (difference between SBP and DBP). Important to note is that the mean arterial pressure (MAP, in Eq. 26.1 ) represents the area under the waveform curve. It has traditionally been felt that the overall magnitude of the reading remains the same regardless of the location of the tracing. However, some evidence exists that, among critically ill adults, MAP readings from radial artery catheters are consistently lower than those from femoral artery catheters by up to approximately 5 mm Hg.
The appearance of the arterial waveform also provides clinical information to the observer. Pulsus alternans ( Fig. 26.6 A) is observed when regular variations occur in the amplitude of the peak systolic pressure during sinus rhythm. This phenomenon can be seen in patients with severe left ventricular failure. Pulsus paradoxus ( Fig. 26.6 B) demonstrates an exaggerated decrease in the systolic pressure (>10 mm Hg) during the inspiratory phase of the respiratory cycle. This phenomenon can be observed in patients with pericarditis, pulmonary hyperinflation, and decreased intravascular volume.

The physiology that leads to pulsus paradoxus in pathologic states may also be used to assess for fluid responsiveness in intubated patients by measuring their pulse pressure variation (PPV). A number of studies have shown that, in intubated patients without spontaneous breathing, substantial (>12%–13%) PPV between cardiac cycles during insufflation and expiration have excellent performance in predicting fluid responsiveness. Many modern monitors can now analyze PPV automatically and report the number to the clinician directly. The physiology of cardiopulmonary interactions that underlie this is discussed at length in Chapter 32 . Briefly, during insufflation of a sufficiently large tidal volume (at least 8 mL/kg ideal body weight) right ventricular preload falls dramatically, resulting in a decreased left ventricular preload after a delay of a few cardiac cycles ( Fig. 26.7 ).

Pulmonary artery catheters
History and controversy
In 1847, Claude Bernard described a method for measuring intracardiac pressures in animals by inserting a glass tube in the heart. However, the true pioneers of cardiac catheterization were two other Frenchmen: Jean Baptiste Auguste Chaveau, at that time a veterinarian interested in the relationship between the dynamic motion of the heart and heart sounds, and Etienne-Jules Marey, a physician interested in the physiology of the circulation. In the early 1860s, using techniques adapted from Bernard’s work, Chaveau and Marey inserted a double-lumen catheter into the right atrium of a horse to record phasic changes in intracardiac pressures as they simultaneously recorded the apical impulse.
Right heart catheterization was not considered a safe practice in humans until the early 20th century. In 1929, Werner Forssman, a German surgeon, secretly performed a right heart catheterization on himself. In direct contradiction to his supervisor’s instructions, Forssman inserted a urinary catheter into his own left antecubital vein and then the remainder of the way to his right atrium under fluoroscopic guidance with the aid of a mirror. Forssman performed right heart catheterizations on himself a total of nine additional times without adverse consequences and expanded his findings by demonstrating the feasibility of injecting contrast dye during the procedure. ,
In the early 1940s, Andres Cournand and Dickinson Richards, working at Bellevue Hospital in New York, continued Forssman’s work. They performed right heart catheterization in healthy humans and in those with cardiac failure. In 1956, Forssman, Cournand, and Richards won the Nobel Prize in Physiology or Medicine for their discoveries relating to heart catheterization and pathologic changes in the circulatory system. They were the first investigators to measure pulmonary capillary wedge pressures using cardiac catheterization. ,
In 1953, Lategola and Rahn performed experiments in dogs in which they were the first to use a self-guiding balloon-tipped catheter to measure pressures in the pulmonary circulation. Seventeen years later, Swan et al. at the University of California, Los Angeles, used this technique to assess right heart pressures in humans. In doing so, they brought this methodology to the bedside, where it is still used today.
In the 50 years since Swan and Ganz added balloon flotation and thermodilution to the PAC, its use has been controversial. The literature is rife with studies reporting salutary effects of placing them, studies showing no effect, and studies showing harm. Due to this controversy and the significant risks attendant to its use, the PAC has fallen out of favor; its routine use today is rare outside of specific clinical scenarios discussed later.
With regard to pediatric patients, the Pulmonary Artery Catheter Consensus Conference, based on a consensus of expert opinions, concluded that the PAC was useful for clarifying cardiopulmonary physiology in critically ill infants and children with pulmonary hypertension; shock refractory to fluid resuscitation and/or low-to-moderate doses of vasoactive medications; severe respiratory failure requiring high mean airway pressures; and, on rare occasions, multiple organ failure. They found no data indicating that PAC use increases mortality in children; however, they also failed to find any controlled trials that demonstrated a benefit of PAC use. The panel recommended PAC use for selected patients and called for randomized controlled trials, a registry of PAC use, and studies to assess the impact of PAC use on cost and duration of ICU/hospital stay. A further review of current studies demonstrated level B and level C evidence for most indications.
Indications
Although controversial, current indications for PAC use in children include septic shock unresponsive to fluid resuscitation and vasopressor support, refractory shock following severe burn injuries, congenital heart disease (CHD), pulmonary hypertension, , multiple organ failure, liver transplantation, and respiratory failure requiring high mean airway pressures. ,
Capabilities of PACs include determination of CVP, pulmonary artery pressure, and pulmonary artery occlusion pressure (PAOP), also referred to as pulmonary capillary wedge pressure. PAOP is a measurement of left atrial pressure and left ventricular end-diastolic pressure (when the mitral valve is open). PACs are also used to assess CO, Sv o 2 , oxygen delivery (D o 2 ) and consumption (V o 2 ), and pulmonary vascular resistance (PVR) and systemic vascular resistance (SVR). The PAC is the only bedside tool that can examine the function of the right and left ventricles separately aside from echocardiography, which provides significantly less precise assessments. PACs are used to establish diagnoses, guide response to therapy, and assess the determinants of oxygen delivery. PACs are especially helpful in cases of discordant ventricular function.
One of the most common uses of the PAC in infants and children is monitoring pulmonary pressures during and after repair of CHD. In addition to flow-directed, balloon-tipped PACs, transthoracic left atrial catheters are often used in these patients. Use of PACs has altered the management of children with CHD by identifying residual anatomic defects and diagnosing pulmonary hypertensive crisis. , The ability to monitor PAP provides the means to titrate response to inhaled nitric oxide and other pulmonary vasodilators. , The lack of response to inhaled nitric oxide may suggest a residual structural anomaly in postoperative patients and indicate the need for interventional cardiac catheterization and/or surgical repair. In addition to monitoring for pulmonary hypertensive crisis, PACs can be used to assess the effects of changes in concentration of inspired CO 2 on mean pulmonary artery pressure (MPAP), pulmonary vascular resistance index (PVRI), and CI.
Monitoring techniques with the pulmonary artery catheter
The functional features of the PAC are several. Its use as a method of indicator thermodilution, measurement of cardiac output, monitoring, and blood sampling is well documented. Fig. 26.8 demonstrates the expected waveforms as the catheter passes through the cardiovascular system. Technical concepts are important for understanding the calculations needed for optimum use. See Table 26.1 for a summary of the hemodynamic parameters that can be derived from a PAC.
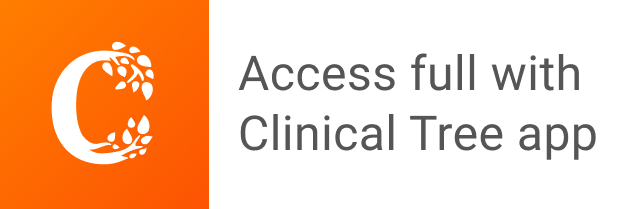