Pearls
- •
Three pathways to breathing failure are impaired neural control, failure of the muscles of breathing, and dysfunction of the mechanics of breathing.
- •
Dysfunction of the mechanics of breathing can contribute significantly to respiratory muscle workload, predominantly through imposition of inefficiencies. Assisted ventilation can (1) prevent breathing failure from progressing to respiratory arrest, (2) improve gas exchange, and (3) reduce metabolic expenditure for muscle work in the patient with limited reserves.
- •
Patients with neuromuscular or respiratory control disorders often do not exhibit typical clinical signs of respiratory distress and may have indolent respiratory failure.
- •
Infants have a number of mechanical disadvantages in breathing that predispose to breathing failure and respiratory arrest.
- •
Multiple causes of breathing failure may occur concomitantly and may be synergistic in causing respiratory failure.
- •
Recognizing impending respiratory failure allows institution of mechanical respiratory support prior to frank respiratory arrest.
Physiology of breathing
Respiration involves movement of air (breathing), diffusion of gases between alveolus and pulmonary circulation, circulation of blood between tissue and lung, and tissue energy metabolism. This chapter provides an overview of spontaneous breathing and breathing gone awry, setting the stage for later chapters on respiratory disorders. The term breathing failure is used in this discussion to limit consideration to failure of the respiratory pump that drives air movement. Breathing failure is arguably the most common cause of arrest in infants and children.
Normal breathing requires neural control to maintain airway patency and drive the muscles of the respiratory pump. Powerful neural regulation of breathing maintains a constant supply of oxygen to the tissues, despite wide variations of metabolic rate and respiratory system disorders, until an advanced stage of respiratory failure is reached. Each of these components of breathing may be deranged in isolation or in combination to produce disordered breathing and, ultimately, breathing failure. The physiology under normal and stressed circumstances leading to these clinical states is discussed in this chapter.
Controls of breathing
Several neural inputs regulate the drive of the respiratory pump and the muscles that maintain airway patency. Derangements of respiratory controls may be the primary cause of acute respiratory failure or one of multiple causes in a critically ill or injured patient. In other patients, disorders of respiratory regulation prolong dependence on mechanical ventilation. Disorders of respiratory controls may be difficult to distinguish from, and may combine with, muscle failure and altered respiratory mechanics.
Metabolically produced carbon dioxide (CO 2 ), and the resultant change in pH drive breathing through central chemoreceptors. The retrotrapezoid nucleus (RTN) within the ventral medulla senses partial pressure of carbon dioxide (P co 2 ) through its effects on local pH and stimulates respiration. , The RTN, in turn, innervates the pre-Bötzinger complex of the ventrolateral medulla oblongata in addition to other brain regions, which generates the rhythmic discharges whose timing corresponds to inspiratory and expiratory phases of respiration. , During resting inspiration, efferent activity stimulates diaphragm flattening. Increased pharyngeal muscle tone and vocal cord abduction act to keep the upper airway patent, allowing inspiratory airflow. During expiration, inspiratory muscles relax. Resting expiration is passive, driven by elastic recoil of the lung and chest wall.
The respiratory rate, inspiratory time, and motor intensity are modified by a variety of rostral neural, chemical, and mechanical stimuli ( Fig. 44.1 ). With high-intensity stimulation, accessory muscles of breathing are activated, including intercostal and neck muscles. Nasal flaring also occurs. Both enhance inspiratory airflow. Stimulated breathing also may include end-inspiratory (premature) vocal cord closure that prolongs lung inflation with little energy expenditure, which is clinically manifested as grunting. In highly stimulated breathing, abdominal muscles contract to force expiratory airflow.

Other normal respiratory control behaviors include periodic deep inspirations, sneezing, and coughing to maintain expansion of basal lung areas and clear secretions from the respiratory tract. Breathing is normally coordinated with swallowing, vocalizing, and airway protective reflexes to prevent aspiration. In extreme circumstances, pharyngeal muscle tone may be adjusted with neck flexion and extension to maintain airway patency.
Peripheral chemoreception in the carotid body modulates the ventilatory response and provides powerful stimulation in response to hypoxemia. Peripheral chemoreceptor activity and ventilation increase slightly as partial pressure of arterial oxygen (Pa o 2 ) falls below 500 mm Hg. However, ventilation rises steeply as Pa o 2 falls below 50 mm Hg and acts as a ventilatory stimulus ( Fig. 44.2 A). Furthermore, low arterial oxygen tension, rather than low oxygen content, is the ventilatory stimulus. This is supported by the observation that there is little carotid body response with profound anemia alone. Hydrogen ion concentration and CO 2 tension independently activate chemoreceptors in the carotid body and brainstem ( Fig. 44.2 B). The simultaneous presence of hypoxia augments the hypercapnic ventilatory response ( Fig. 44.2 C).

Mechanical loads on breathing influence respiratory efforts immediately, independent of chemical stimuli. Sensors for load-compensating reflexes are located in respiratory muscles and the chest wall. Reduction in lung volume is also detected by pulmonary stretch receptors. Afferent signals from loaded breathing travel via the spinal cord, vagus nerves, and perhaps the phrenic nerves. Both conscious and reflex responses are involved in compensatory increases of effort, including the recruitment of accessory muscles in response to increased respiratory resistance or to a decrease in compliance. Stimulation to breathe is further augmented by hypercapnia or hypoxemia when loaded breathing reduces ventilation. Mechanical loads to breathing, and the resultant afferent stimuli, can actually promote hyperventilation and hypocapnia. Dyspnea and anxiety exacerbate the tendency to hyperventilate even without a chemical ventilatory stimulus.
Respiratory pump
The respiratory pump comprises the diaphragm, the intercostal muscles, and the accessory muscles of breathing. Each contributes to breathing to a varying degree depending on the neural input and respiratory load.
Diaphragm
The diaphragm arises from the embryologic pleuroperitoneal fold. Myoblasts migrate from cervical somites to the pleuroperitoneal fold, where they arrange themselves into a sheet on a mesenchymal substrate that separates the peritoneum from the abdomen. Once fully formed, the diaphragm originates from bilateral tendinous crura attached to the spinal column and inserts as a costal tendon attached to the chest wall between the sixth and twelfth ribs. The dome of the diaphragm remains largely tendinous. This configuration, a circular attachment to the thoracic wall, vertical muscle orientation adjacent to the thorax, and attachment to a flattened central tendinous dome ( Fig. 44.3 ), works like a piston during breathing to enlarge the thorax and displace abdominal contents downward.

Approximately 50% of the diaphragm consists of type I fast-twitch muscle fibers, which have high endurance and are resistant to fatigue. The remainder of the diaphragm is made up of type IIA and type IIB fibers, which have different properties. Type IIA fibers are important in achieving high levels of minute ventilation quickly, have good endurance, and can contract rapidly, but they are unable to sustain long-term power output. Type IIB fibers cannot sustain their force of contraction because they possess lower oxidative capacity and are more susceptible to fatigue. The greater the force of contraction required, the more motor units of the diaphragm are recruited. There appears to be little difference between the activity of costal muscles and crural muscles, either during normal breathing or in response to hypoxia and hypercapnia.
When a patient lies supine, the diaphragm rests against the inner surface of the rib cage. When the diaphragm contracts and its muscle fibers shorten, the whole diaphragm moves down, lowering pleural pressure and increasing intraabdominal pressure. The increase in intraabdominal pressure generated by descent of the diaphragm acts as a caval pump to enhance cardiac filling. Because of its alignment against the lower ribs (zone of apposition), descent of the diaphragm also expands the caudal portion of the rib cage. The muscle of the diaphragm extends from the costal insertion to the dome of the diaphragm. When the diaphragm is “high,” it is loaded for greater force of contraction. When it is “low” or “flat,” it is unloaded and mechanically disadvantaged.
The diaphragm is the major inspiratory muscle of the neonate. It increases in thickness with age (as its muscle mass increases). It also becomes appositional to a longer segment of chest wall with growth, enhancing its effectiveness as an inspiratory piston. In the neonate, the muscular diaphragm has a greater angle from the vertical than that of the adult, which reduces its effectiveness as an air pump ( Fig. 44.4 ). This angle approaches zero with growth, increasing the diaphragm’s effectiveness with advancing age. The importance of this angle as an impediment to diaphragmatic effectiveness becomes exaggerated at total lung capacity, with air trapping, and when the abdomen is distended, all of which flatten and unload the diaphragmatic muscle ( Fig. 44.5 ).


Intercostal muscles
The rib cage is fixed to the spine and sternum. The spine maintains a relatively fixed separation between adjacent ribs. Vertical mobility of the sternum allows lift and descent of the anterior rib cage. Thoracic volume is modified during breathing primarily by changing the angle of the anterior ribs to the horizontal. At rest, the ribs slope caudad from their spinal attachments. The rib cage tilts upward during inspiration. The intercostal muscles form three functional sheets. The outermost (external) sheet and the parasternal sheet act to displace the ribs cephalad as they shorten. This increases both the anteroposterior and lateral dimensions of the thorax. There is also a deep (internal) layer of intercostal muscle at right angles to the external sheet that acts to displace the ribs caudad when it contracts. Thus, the intercostal muscles play both inspiratory and expiratory roles in breathing by reshaping the thorax.
Accessory muscles of respiration
Though quiet expiration is largely passive, resulting mostly from inward elastic recoil of the lung, active expiration may be assisted by contraction of abdominal muscles (rectus abdominis, transverse abdominis, and the obliques). During quiet breathing, abdominal muscle tone elevates the diaphragm during expiration, increasing the zone of apposition and loading the diaphragm for greater inspiratory contractile efficiency.
Accessory muscles of inspiration include the scalenes, sternocleidomastoids, pectoralis minor, and erector spinae, all of which elevate the ribs during contraction. During quiet breathing, accessory muscles play a minor role, but during respiratory exertion, they may play a major role and act to unload and unburden the diaphragm and intercostals. Even profoundly neurologically impaired children, who exhibit little volitional activity, can use accessory muscles of breathing when distressed. Children with dysfunction of the primary muscles of respiration, such as with inherited myopathies or denervation, may rely on their accessory muscles even at rest.
Breathing failure
Several discrete mechanisms may cause breathing failure ( Table 44.1 ). Each of these final common pathways may be triggered or compounded by other discrete adversities, and independent pathways of breathing failure may converge to cause respiratory arrest (or need for mechanical support of breathing).
Mechanism | Examples |
---|---|
Failure of neural control | Uncal herniation, central hypoventilation |
Failure of muscles of breathing | Insufficient muscle blood flow, hypoxemia |
Failure of mechanics of breathing | Flail chest, diaphragmatic paralysis |
Failure of respiratory controls
Disorders of respiratory controls may present with impaired respiratory cycle generation (central apnea), deficient responses to respiratory stimuli (hypoventilation during stress and failure to arouse from sleep hypoxia), or inadequate motor control of the vocal cords or pharynx (causing stridor or stertor). These patterns of regulatory dysfunction may occur individually or in combination.
Recognizing depressed respiratory drive
Respiratory neural output is not routinely directly measured in the clinical setting. Interpreting ventilation as a measure of the intensity of drive to breathe is confounded by dependence of ventilation on the multiple factors of muscle strength, respiratory system mechanics, and respiratory neural motor output. Nevertheless, the stressed patient who appears inappropriately comfortable, with little accessory muscle activity, or with periodic breathing, should be presumed to have depressed controls of breathing warranting assisted ventilation.
Failure of neural control
Rhythmic control of breathing by the central nervous system may fail for a variety of reasons. The brain is susceptible to injury, ischemic stroke, central nervous system hemorrhage, suppression of brain function by cold, structural dysfunction, chemical suppression, signal disruption, and inherited disorders of respiratory control. Efferent neural pathways may be blocked by spinal cord lesions, spinal anesthesia, phrenic nerve injury, or neuromuscular disorders. Examples are cited in Table 44.2 . Note that volitional and automatic control of breathing may be separately and independently affected. In Ondine’s curse (resulting from stroke involving the lateral medulla), automatic control is impaired, whereas volitional control may persist in the awake state. In contrast, supratentorial stroke may impair volitional control of breathing, though automatic control of breathing may be preserved.
Mechanism | Example |
---|---|
Brain injury | Closed head trauma |
Ischemic stroke | |
Severe supratentorial stroke | Locked-in syndrome (loss of volitional control of breathing) |
Lateral medullary stroke | Ondine’s curse (loss of automatic control) |
Subarachnoid hemorrhage | Intracranial hypertension with uncal herniation |
Brain suppression by cold | Cold-water drowning |
Structural brain dysfunction | Brainstem glioma |
Chemical suppression | Narcotic overdose |
Signal disruption | Seizure |
Intrinsic Disorders | |
Idiopathic | Ondine’s curse |
Immaturity | Apnea of prematurity |
Spinal cord lesion | Poliomyelitis, traumatic cord transection |
Spinal anesthesia | Inadvertent spinal anesthesia |
Phrenic nerve injury | Cardiac surgical phrenic nerve injury |
Neuromuscular disorder | Curare poisoning, botulinum, and many snake venoms |
Central hypoventilation is abnormal respiratory control by the brain and results in hypercapnia. Patients with central hypoventilation may have otherwise normal neurologic functioning or they may have concomitant neurologic injury. Unless mixed with peripheral neuromuscular disease, patients have normal muscle strength, but the essential feature is altered responses to respiratory acidosis. Respiratory patterns may also be abnormal. Central hypoventilation is often most apparent when patients are sleeping. Apnea of prematurity, which may persist beyond term, is still not well understood but is clearly aggravated by concomitant infection.
Peripheral (extracranial) neural disorders include disorders of the anterior horn cells (e.g., spinal cord injury, spinal muscular atrophy, infections), disorders of the peripheral nerve (e.g., Guillain-Barré syndrome), disorders of the neuromuscular junction (e.g., myasthenia gravis, anticholinesterase poisoning, botulism) and disorders of muscle (e.g., Duchenne muscular dystrophy, congenital myopathies). The hallmark of these diseases is muscle paresis or paralysis. Hypercapnia is a common feature. Depending on the extent of weakness, patients will be unable to increase their work of breathing in the face of lung disease. Importantly, clinical signs such as tachypnea and accessory muscle usage cannot be relied on. Abnormal arterial blood gases may be the only indications of worsening breathing failure, especially in patients with altered sensorium.
Patients with spinal cord lesions develop muscle weakness below the level of injury. For instance, patients with lower cervical cord injury may have preservation of suprasternal muscle action but have loss of other accessory muscle function as well as diaphragmatic dysfunction or paresis. These patients will have vigorous suprasternal retractions that expand the lung apices but have little other movement.
Structural brain lesions
Moderate brain injuries are typically associated with hyperventilation, whether the injury is traumatic, infectious, or hypoxic-ischemic. The hypermetabolic state, lung pathology, and loss of inhibitory cortical influences probably combine to augment ventilation. Even when the brain-injured patient does hyperventilate, airway protective reflexes are usually impaired, seizures may ensue, and subtle progression of the brain lesion may lead to hypoventilation. Resulting hypoxia exacerbates the brain injury.
Nonstructural acquired disorders
Seizures impair breathing in various ways. Apnea or slowing of respirations, impairment of upper airway protective reflexes, and poor inspiratory effort are common. Because infants can have subtle manifestations of seizure, the clinician must have a high index of suspicion to recognize occult seizures as a cause of hypoventilation. The seizure-induced respiratory depression may be difficult to distinguish from the brain pathology predisposing to the seizure (e.g., infection, hemorrhage) as well as the respiratory-depressing effects of anticonvulsant medications.
Respiratory depression by analgesic, sedative, anticonvulsant, and anesthetic agents is common in the pediatric intensive care unit. Relative effects on upper airway patency and hypoxic, hypercapnic, and loading responses may be dissociated. Concern regarding respiratory depression does not warrant withholding analgesia, however, but rather warrants appropriate monitoring. In fact, the patient experiencing pain may breathe shallowly, exacerbating hypoxia. Sedatives and analgesics that are rapidly cleared after a single dose may have a more prolonged duration of action when given repeatedly or continuously. Clearance rates for medications may also vary with systemic disease, immaturity, or genetic factors. Sedation and analgesic-induced respiratory depression may unnecessarily prolong the need for mechanical ventilation.
Opioid-induced respiratory depression can be reversed with naloxone. However, in patients with cardiovascular compromise (e.g., those who have had cardiac surgery), naloxone should be avoided in the immediate postoperative period because abruptly eliminating analgesia can provoke dangerous increases in catecholamines. In the patient with multiple drug ingestions, naloxone may induce vomiting without improving airway protective reflexes, predisposing the patient to aspiration. The benzodiazepine antagonist flumazenil reduces the respiratory depression that results from taking benzodiazepines, but little pediatric experience with this agent has been reported. Flumazenil lowers the threshold for seizures and may cause a more hazardous condition than the initial respiratory depression. Importantly, the duration of action of antagonists is often shorter than the agent that is depressing breathing. Close monitoring of the patient is essential, and repeated doses of antagonists may be necessary. In other cases of drug-induced respiratory depression, mechanical ventilation provides greater safety than do pharmacologic antagonists, particularly with multifactorial central depression or in severely ill patients.
Other medications may depress breathing without alteration of consciousness. For example, prostaglandin E1, which is given to maintain patency of the ductus arteriosus in infants with congenital heart disease, is frequently associated with respiratory depression. Metabolic alkalosis, which may result from diuretics and other medications, can promote respiratory depression and may contribute to prolonged dependence on mechanical ventilation. When metabolic alkalosis accompanies prolonged recovery from respiratory failure, correction of the alkalosis may promote ventilator weaning.
Chronic respiratory failure
Congenital or long-standing acquired disorders of the central nervous system may impair respiratory centers, leading to respiratory failure. Acute respiratory insufficiency may accompany progression of a central lesion. Alternatively, static regulatory impairment may be revealed by failure to compensate for acute systemic illness.
Structural brain lesions
Recognition of structural brain lesions as the cause of impaired respiratory regulation is important because some of the lesions are correctable. Congenital structural neurologic malformations may manifest as apnea or profound hypoventilation at birth or may be recognized later. In particular, patients with Chiari malformations often have central and obstructive sleep apnea. Surgical decompression may be associated with improvement even when performed in adults. Acquired lesions, such as posterior fossa tumors, may interfere with respiratory regulation before or after surgical resection.
Nonstructural congenital disorders
Some genetic conditions are associated with derangements in regulation of breathing. Children with congenital central hypoventilation syndrome have characteristic mutations in the PHOX2B gene, which is critical for function of the retrotrapezoid nucleus. These patients may first come to medical attention because of growth failure, neurodevelopmental disabilities, or cor pulmonale. Other abnormalities include autonomic dysfunction and cardiovascular instability, occasional association with Hirschsprung disease, tumors of neural crest origin, and impaired controls of breathing. The syndrome may be recognized in the newborn period, later in childhood, and rarely in adults. Sleep hypoventilation predominates in persons with congenital central hypoventilation syndrome, although some patients also experience respiratory insufficiency while awake. The disorder is often fatal without mechanical ventilation. Early mechanical ventilation may reduce the sequelae and improve long-term neurodevelopmental outcome.
Prader-Willi syndrome is a multigenic disorder initially presenting with hypotonia and then with progressive obesity, growth failure, neurodevelopmental disabilities, reduced ventilatory response to hypoxia and hypercapnia, sleep hypoventilation, and apnea.
Rett syndrome is an X-linked disorder affecting development, behavior, autonomic and respiratory regulation, and seizures. Occurring only in girls, most cases involve spontaneous mutations, although occasionally other family members are affected. Abnormalities are often present in the MECP2 gene, although some patients with characteristic clinical features have other genetic findings. Multiple phenotypes exist in regard to the respiratory control disorder, with hyperventilation, hypoventilation while awake or asleep, and apneustic breathing.
Many other genetic syndromes with severe neurologic manifestations have impaired upper airway motor function, respiratory cycle timing, and respiratory effort nonspecifically associated with their brain disorder. Nongenetic congenital disorders may impair respiratory controls. For example, children with cerebral palsy occasionally have neurologic deficits of pharyngeal tone, although the central drive to breathe is usually intact. For this reason, these patients may paradoxically have improved ventilation with sedation during times of significant respiratory distress caused by noxious stimuli.
Nonstructural acquired chronic disorders
Some patients with severe chronic respiratory disease have blunted ventilatory responses to hypoxia, hypercapnia, or respiratory mechanical loads. A concern regarding supplemental oxygen is sometimes raised in the care of patients with acute exacerbations of chronic respiratory disease. It is sometimes argued that administration of supplemental oxygen causes respiratory failure in patients with chronic CO 2 insensitivity who might depend on hypoxic drive to breathe. However, the adverse effects of hypoxia are a greater concern. Because the hypoxic drive to breathe increases substantially only at oxygen tension below 50 mm Hg (see Fig. 44.2 A), it is virtually impossible to maintain stable respiratory stimulation with mild, “safe” hypoxia without risking episodic life-threatening episodes. If a patient is so poorly compensated that removal of hypoxic drive results in hypoventilation, then mechanical ventilation may be the safest management strategy unless end-of-life plans specifically exclude mechanical ventilation.
Several clinical conditions are commonly associated with hypoventilation. Obesity causes hypoventilation by a complex interaction of factors, including mechanical loads on the respiratory system, reduction of lung volume, upper airway obstruction, and impaired respiratory regulation. In obese patients, weight loss often improves hypoventilation. Apparently healthy preterm infants may have postanesthetic apnea until the age of 60 weeks’ postconceptional age. , Apneic events occurred within 2 hours of surgery in 72% of patients, but in the remainder, respiratory irregularity began as late as 12 hours postoperatively. Both obstructive and central mechanisms of apnea were observed. Continuous monitoring for at least 12 hours after anesthesia is warranted when surgery is required for infants born prematurely who are still younger than 60 weeks’ postconceptional age. Sleep hypoventilation tends to occur in adults with hypothyroidism and diabetes mellitus. Little information is available regarding the pediatric patient or the specific role of the endocrine disorder versus the effect of obesity.
Respiratory pump failure
Causes of respiratory pump (breathing muscle) failure are listed in Table 44.3 , along with examples. They may be crudely divided into direct causes of muscle failure (plegia, paralysis, and tetany) or muscle failure from exhaustion. Exhaustion may result from impaired delivery of substrate to the muscles or from increased load due to increased ventilatory demands, or mechanical failure of the respiratory system, including restrictive or obstructive lung disease. In most cases, patients progress through a typical phase of compensation based on the mechanism of the insult, allowing clinicians to identify and attempt to intervene before frank breathing failure.
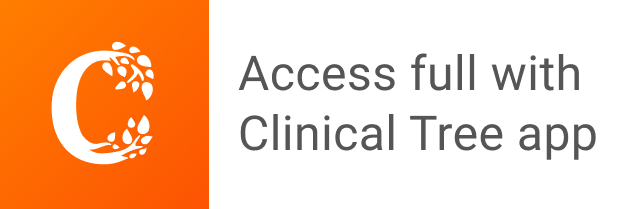