Optimal and Safe Use of C-Arm X-Ray Fluoroscopy Units
- Peter J. Mas
Introduction
The use of fluoroscopic imaging procedures allows healthcare professionals to view the examination and procedure of the patient in real time. Modern fluoroscopic devices are greatly improved from the darkened room imaging conducted in the mid-twentieth century. The imaging system or image intensifier assembly is the fundamental component for the production of the patient image. It has grown from an approximately 6-inch-diameter image intensifier to a 12-inch-diameter device on mobile C-arm units. Stationary C-arm fluoroscopic units, such as those found in a busy radiology department, can come equipped with an 18-inch image intensifier, although it is more common to see a 15-inch intensifier ( Fig. 13A-1 ).

The term C-arm was coined because of the letter C-shaped yoke of the x-ray tube-to-image intensifier geometry ( Fig. 13A-2 ).

In addition to the x-ray production electronics, display electronics, the x-ray tube and collimation device, the C-arm unit also has an antiscatter grid, a charge-coupled device (CCD), and a video system camera to feed the image onto the display monitors. Mobile C-arm units are equipped with wheels and a steering mechanism for transport to the procedure room or operatory where it will be used.
Features
The image intensifier assembly is composed of:
- 1.
An antiscatter grid (reduces scattered x-rays entering the unit)
- 2.
A vacuum tube with:
- a.
Photoabsorptive and electroemissive surfaces
- b.
Electrostatic focusing electrodes
- c.
An output phosphor (CCD)
- a.
- 3.
Light-focusing lenses, diaphragm, and video signal pickup
- 4.
Electronic shielding
- 5.
Lead-lined enclosure (serves as the primary x-ray barrier)
The function of each component of the image intensifier system is summarized below.
- 1.
Antiscatter grid: Reduces the loss of image resolution due to scattered x-rays reaching the image intensifier ( Fig. 13A-3 ). Scattered x-rays are those deflected from the original “straight-on” path to the image intensifier.
Figure 13A-3
The complete image intensifier assembly.
- 2.
Vacuum tube: Made of glass or a nonferromagnetic material ( Fig. 13A-4 ). Provides for the accelerated travel of electrons emitted from the entrance (input phosphor-to-photocathode) surfaces and directed to the output phosphor at the opposite end.
Figure 13A-4
Output phosphor electronic shielding.
- 3.
Electrostatic focusing lenses: Changing the electrical bias on these lenses will spread out (or compress) the stream of electrons coming from the photocathode surface, thereby causing a magnification (or minification) of the resultant image being captured.
- 4.
Output phosphor: Produces light photons representative of the x-rays absorbed and transmitted within the patient. ( Fig. 13A-5 )
Figure 13A-5
The output phosphor of the image intensifier.
- 5.
Video signal pickup: The image generated at the output phosphor is then viewed with the attached video system (TV camera or CCD). An Automatic Brightness Control (ABC) feedback circuit is used to drive the x-ray generator to produce more (or less) x-rays by increasing (or decreasing) the x-ray tube kVp operating potential or the x-ray tube mA current.
- 6.
Electronic shielding: Used to reduce any possible distortion of the image production system from external sources of electric and magnetic fields
- 7.
Lead-lined enclosure: The image intensifier system is a primary radiation barrier. X-rays do not escape out of the image intensifier back into the suite. ( Figs. 13A-6 and 13A-7 ).
Figure 13A-6
Lead-lined housing of the image intensifier.
Figure 13A-7
View of the interior.
I have not given much attention to the x-ray tube itself, primarily because its purpose is limited: it either produces x-rays or not. The x-ray tube does not independently manage, manipulate, or modify the x-rays produced, but the x-ray tube housing is another matter. The tube housing ( Fig. 13A-8 ) is a lead-lined enclosure with three important components of the x-ray generation chain in addition to the x-ray tube itself: the x-ray beam filter(s), the beam collimation (an x-ray field size-limiting device), and a thermal switch to sense overheating of the x-ray tube.

X-rays are produced when a stream of electrons are accelerated toward a high atomic (Z-number) target material, such as tungsten, functioning as the anode in an electrical circuit. The collisions of the electrons with the target results in x-rays, but approximately 99% of the collisions simply result in the heating of the target, and a thermal overload interrupt switch becomes a necessity. The tube housing also serves as a barrier against x-rays. By Food and Drug Administration (FDA) regulation, the x-rays escaping the tube housing (“leakage x-rays”) cannot result in a radiation exposure rate greater than 0.1 roentgen per hour, measured at 1 m from the x-ray source, when operated at its maximum kVp energy and maximum continuous mA tube current.
X-rays produced in this manner are termed “polychromatic” because they cover a wide spectrum of energies; they are not monoenergetic like gamma-ray (nuclear) sources of radiation. The addition of x-ray beam filters achieves a cleaner and higher effective energy x-ray beam profile. The insertion of aluminum metal filters will remove (filter out) the lower energy x-rays, which cause much greater (entrance-skin surface) radiation exposure to the patient and do not contribute to the creation of the diagnostic image. The higher energy x-rays continue to pass through the added aluminum filtration and reach the patient for the selective attenuation by tissues and anatomic structures. X-rays exiting the patient can now interact with the image intensifier input phosphor, and the imaging process commences. Higher energy x-ray imaging devices, such as computed tomography (CT) scanners, use copper metal beam filtration to essentially eliminate all low-energy x-rays.
The x-ray beam collimation component is also governed by FDA regulation. The x-ray beam can never be larger (wider) than the image intensifier diameter, and the image intensifier must be affixed to the x-ray device in such a way that it always intercepts the x-ray beam. An unattenuated, primary beam x-ray field must never extend beyond the physical size of the x-ray primary barrier that is built into the image intensifier device. In the clinical setting, such a misalignment would be comparable to standing next to a bulls-eye while the projectiles are hitting outside of the intended target. The manufacturer can place a lead diaphragm (or cone) at the x-ray tube to satisfy the beam-size limiting criteria, but physical abuse or mishandling of the unit may compromise the x-ray beam-to-image intensifier alignment.
The final components of the imaging system are the x-ray control panel ( Figs. 13A-9 and 13A-10 ), the exposure activation switches, customarily performed via a “dead man” type foot switch ( Fig. 13A-11 ), and the image display and recording device ( Fig. 13A-12 ). The x-ray control panel indicates the mode of operation of the C-arm unit. Details such as the kVp beam energy, the mA tube current, the elapsed time for the procedure, the timer alarm reset, the imaging frame rate, the intensifier MAG (magnification) mode, and image display parameters are viewed and controlled at the console. Nearly every one of these has a direct bearing on the patient’s (and staff) radiation exposure, which will be described in a later section.




The “dead man” foot switch is so called because you must actively depress the switch to get the x-ray beam “on.” If the operator were to drop dead during the procedure, the spring-loaded foot switch would return to the “off” position, the C-arm unit would drop to zero x-ray emissions, and it would again be “radiation safe.” The foot switch is on a long cord for placement where most convenient to the physician or operator of the C-arm.
The image display and recording and archival device is the final leg of the diagnostic imaging process. The monitors must be of sufficient resolution and brightness to clearly display the progress of the procedure. Most C-arm units sold presently include an integrated hard drive with picture (frame) grabber hardware and software. The stored images can be called up for review and printing onto film media, but there is a finite number of images that can be stored. Depending on the hard drive capacity, that number ranges from a low of 100 to as much as 10,000, and when the storage media has been filled, the unit will write over the first image in storage and continue therefrom. The advantage of a “last image hold” frame grabber is to see the last recorded position of the device(s) you are manipulating or inserting into the patient on the monitors. Therefore, you do not need to maintain the x-ray beam “on” at all times to review your progress in the procedure.
Reduction of Radiation Dose during C-Arm Operation
No two patients are exactly alike. There will be differences in size, height, weight, general shape, and anatomy, but a properly operated mobile C-arm unit will do quite well for the vast majority of cases.
Patients are routinely imaged on an operating room table, a procedure table, a stretcher, and even in a hospital bed. When you have the ability to select what surface the patient will be imaged on, it is better to select an “x-ray compatible” table surface, plus its mattress or foam padding. The designation of x-ray compatible means that the table material itself is not highly attenuating of the x-ray beam. Attenuation of the x-rays results in:
- 1.
Loss of object contrast. The C-arm unit will be forced to operate at a higher imaging technique (greater kVp beam energy or higher mA tube current) as a result of the ABC feedback circuit. The ABC compensates for the reduced light level from the output phosphor caused by the attenuation of the x-ray beam by the table materials. The imaged object contrast (discernible shades of gray) decreases with increasing x-ray energy.
- 2.
Greater entrance skin exposure to the patient from the increased imaging technique
- 3.
Greater amount of scattered radiation during the procedure
The most desirable, but also expensive, surface is the carbon-fiber tabletop. It is the least attenuating because of its low atomic (Z-number) material composition.
A few words about “scattered radiation,” the how and the where it occurs with a C-arm unit. How best to spatially describe the concept of scattering? I like to use the following analogy. Consider driving a car on a clear night. The headlights shine and easily illuminate the road ahead. Now picture yourself driving that same car on a foggy night. There is a “halo” or “corona” of light starting at the headlight surface and radiating outward but generally in the direction of the road surface. You have just witnessed “scattered” radiation, but in this example, the radiation is in the form of visible light that is being diffracted by the water molecules suspended in the air. X-rays can be scattered when striking air molecules, but the denser the material it interacts with, the greater the probability for scattering (and absorption) of the x-rays ( Figs. 13A-13 and 13A-14 ).


Diagnostic imaging x-rays are a low-energy form of electromagnetic radiation. For such, the scattering of the kilovoltage x-rays is predominantly directed back toward the x-ray tube. In comparison, high-energy megavoltage x-rays such as those used in cancer treatment will scatter forward beyond the patient and continue in the direction of the treatment x-ray beam. From a radiation protection perspective, when the C-arm is positioned more parallel to the floor (shooting across the procedure table), personnel should not stand close to the x-ray tube. It is better to stand at the image intensifier side, where there is less scattering of the x-rays originating from the patient.
We now move ahead to when the C-arm is brought to the patient’s side while undergoing the orthopaedic procedure. The next exposure reduction step starts with the positioning of the C-arm at the patient. The single most important positioning criterion to try to adhere to is place the image intensifier as close as possible to the patient’s body ( Figs. 13A-15 and 13A-16 ). Use a sterile cover on the intensifier to maintain the sterility of the operatory field. In this orientation, you achieve three things in your favor:
- 1.
Increased sensitivity to the exiting x-rays that generate the patient’s image plus decreased scattered radiation because the image intensifier is a leaded barrier that has been placed nearer to the body that causes the scattering. When the intensifier is positioned closer to the patient, there is less scattering of x-rays beyond it into the room environment.
- 2.
Increased image resolution. The improved imaging geometry will increase image sharpness, much like a hand will cast a sharper shadow the closer it gets to a wall or the floor (assuming the light is coming from overhead). Here, the hand is the object you need to “see” clearly, and the wall (or floor) is the “imaging” device.
- 3.
Decreased entrance skin exposure at the patient because the x-ray tube is kept the farthest distance possible from the patient’s skin.


Radiation intensity follows the inverse-square law: the change in intensity is proportional to the square of the distance from the source. For example, if you double (factor of 2) the distance away from the radiation source, the intensity drops by the inverse, squared product (1/2 × 1/2), which equals 1/4 (25%) of the original radiation intensity. Conversely, if you halve (1/2) the distance from a radiation source, you quadruple (4) the intensity of the exposure; this is most worrisome whenever you image in a truly lateral orientation. It is best to maintain the x-ray tube as far as possible from the patient’s body surfaces and the image intensifier as close as possible. One FDA-required component of fluoroscopic imaging systems, albeit frequently removed from the C-arm, is the spacer cone (see Fig. 13A-8 ). The spacer cone is used to maintain a 12-inch distance from the patient’s body; otherwise, very large radiation exposure rates and skin doses can be given to the patient. The FDA has publicized warnings to this effect and has identified the procedures most likely to cause conspicuous radiation damage to patients’ skin. Orthopaedic surgical procedures are not on that list, but they can contribute to the deterministic (somatic) effects of skin epilation and skin erythema. For more information, go to the FDA’s website at www.fda.gov/cdrh/rsnaii.html .
Today’s C-arm units have the ability to magnify the area being imaged and are usually designed with two “MAG modes” of operation. Image magnification is achieved within the intensifier by changing the bias voltage on the electrostatic focusing lenses. The electron beam traveling toward the output phosphor is forced to spread out, thereby eliminating some of the signal from the periphery of the electron stream that would have struck the output phosphor. Simultaneously, the collimator assembly narrows down the field of view because less of an area of the patient is being imaged. The resultant effect is less of the image intensifier input phosphor is being irradiated; consequently, fewer electrons are released into the intensifier assembly. The process is reversed when the control panel button for “normal” viewing is pressed.
Let us look at the net effect on the production of the electron stream at the input phosphor with the following images and a bit of algebra ( Figs. 13A-17 to 13A-20 ) for an intensifier of 12–9–6 inch viewing modes. In “normal” viewing mode, a 12-inch circular image intensifier is almost fully irradiated by the x-ray beam exiting the patient; the area (πr 2 ) of that surface equals 36π sq in. At MAG mode 1, the intensifier decreases to 9-in diameter and surface area drops to 20.25π sq in. At MAG mode 2, the intensifier decreases to a 6-inch diameter and surface area drops to 9π sq in. Changing the intensifier size from 12 to 9 inches has a net effect of losing approximately 45% of the input phosphor’s imaging area. Changing the intensifier size from 12 to 6 inches has a net effect of losing approximately 75% of the input phosphor’s imaging area.




The decreased input phosphor area translates directly into a decreased production of electrons inside the image intensifier. The decreased number of electrons results in decreased light production by the output phosphor. The ABC feedback circuit seeks to correct the situation by calling on the x-ray generator to increase the tube energy potential (kVp), the tube current (mA), or both. A higher energy (kVp) x-ray will cause more electrons to be released by interactions at the input phosphor. A higher tube current (mA) will increase the production of x-rays at the x-ray tube. Ultimately, a combination of these two x-ray generator adjustments will satisfy the ABC circuitry. C-arm operation in a magnification modality is one source of significant increases in the imaging techniques (kVp, mA) and will result in:
- 1.
Increased image resolution (at high MAG mode); the patient object will appear larger allowing the viewer to see the smaller details.
- 2.
Increased patient entrance skin exposure because the x-ray tube is operating at a higher technique. If the magnification is doubled (2×) the patient’s exposure rate is quadrupled (4×).
- 3.
Increased heat loading of the x-ray tube. The C-arm may shutdown because of overheating, particularly if performing a lengthy procedure on a larger-sized patient body.
To reduce the exposure of the patient and the personnel and to reduce the heating of the x-ray tube and increase its usable operating time, the magnification mode imaging features should be called upon only when necessary for the task at hand. MAG mode imaging is another tool of the C-arm but one to be used sparingly. Two other tools of the C-arm that impact image acquisition, patient exposure, and image storage are: the “high-dose rate” fluoroscopy mode and the “frame rate” pulsed fluoroscopy settings found at the control panel.
The FDA limit for the patient’s skin entrance exposure rate (at tabletop) is 100 milligray (mGy) per minute (10 roentgens/min in the old terminology). Fluoroscopic examinations typically yield skin entrance exposures ranging from 10 to 100 mGy/min, with 50 mGy/min being a fair estimate for the routine, uncomplicated patient studies. An exception to the limit is the “high-dose rate” feature on fluoroscopic units, including C-arms. This feature is also known as “boost” mode and may be called into use when you encounter an extremely large patient and the normal exposure rate does not produce enough x-rays for the imaging. The high-dose rate feature can go up to 200 mGy/min (20 roentgens/min) skin entrance exposure rate, and a visible and audible alert must be produced by the imaging equipment when operated in this modality. Human skin will suffer epilation from x-rays when it has received radiation exposure as low as 1000 mGy (100 roentgens), and skin erythema occurs with 2000 mGy (200 roentgens) of exposure. These somatic effects take from 1 day to 3 weeks to become evident. You can recognize the value of having a visible and audible alert with an imaging system that can cause 200 mGy/min exposure at skin entrance; this patient may have to cope with radiation damage to the skin at little more than 5 minutes of fluoroscopy time into the procedure at this high exposure rate.
There is another alert located on C-arm devices, the elapsed fluoroscopy time warning bell. The timer routinely alarms at 4 to 5 minutes of elapsed fluoroscopy beam “on” time and has to be reset at the control panel. You can use this as a marker of your progress during the procedure and as a marker of the accrued total skin dose. If you assume 50 mGy/min (5 R/min) skin entrance exposure for a “normal-sized” patient, then 20 minutes of fluoroscopy approaches 1000 mGy.
The acquisition and recording of the fluoroscopic image can be changed at the control panel with the pulsed fluoroscopy feature. In contrast to real-time fluoroscopic images that are captured at a rate of 30 frames per second (33 msec imaging frame time), pulsed fluoroscopy operates with very short exposure times, usually 10 msec per pulse. The outcomes to using this feature are:
- 1.
Reduced patient motion blurring in the acquired image. Picture in your mind the “frozen” and discontinuous images of dancers when a strobe light is pulsing and illuminating the dancers on a dance floor.
- 2.
Reduced total patient and personnel exposures from the reduced amount of x-ray “beam on” time
- 3.
Reduced heat loading of the x-ray tube target, allowing for far longer time of operation in the generation of x-rays
The exposure reduction is proportional to the decrease in the image frame rate. If you drop from 30 to 15 frames per second, you have halved (1/2) the exposures. If you further reduce to 7.5 frames per second, you are at one-fourth (1/4) of the original exposure rate. The drawback lies with the operator; he or she may not like seeing discontinuous images acquired at 15 frames per second and even less so at 7.5 frames per second.
We are accustomed to television video display of 60 frames per second, and real-time fluoroscopy was routinely performed at that imaging rate. This “watching a movie” format gives a sense of fluid motion that is lost at very low image capture rates. With fluoroscopic x-ray imaging, you must realize you are not watching video entertainment . You are observing the gross deposition, absorption, and transmission of radiation energy within a patient. If enough energy is imparted to parts of that living body, you may well cause the appearance of deterministic (somatic) radiation effects to the patient within a short period of time.
A lower frame rate feature is desired when the purpose is to observe the general placement of readily observed medical devices such as orthopaedic screws, electric leads, and some catheters. If you need to assess the progress of your work in a millimeter-by-millimeter fashion or you are imaging a structure with a high degree of motion, you will likely prefer 15 frames per second images.
In Summary: to Operate a C-Arm and Reduce the Radiation Exposure
The essential information in this chapter for the radiation safe imaging of the patient and the dose-limiting exposure to all involved staff, can be reduced to these key elemental points:
- 1.
Minimize the amount of fluoroscopy “on” time. Step off the pedal whenever possible.
- 2.
Use “good geometry” by positioning the patient as close to the image intensifier and as far from the x-ray tube as possible.
- 3.
Reduce the size of the x-ray field (collimate) to view only the anatomical region of interest, thereby reducing the scattering of x-rays and sparing unintended body tissue(s) from the radiation.
- 4.
Minimize use of MAG modes and “boost” (high dose rate) fluoroscopy.
- 5.
Maximize your use and viewing of the “last image hold.” If nothing has changed, rely on an image already obtained as opposed to generating more x-rays.
- 6.
Whenever possible, select the lowest dose rate (combination of highest kV and lowest mA) ABC setting that gives acceptable image quality.
- 7.
Use pulsed fluoroscopy. Use the lowest pulse rate and pulse width (msec) consistent with acceptable temporal resolution.
- 8.
Vary the x-ray tube angle (to the extent possible) to reduce the skin dose to any one area when long fluoroscopy times (>20 min) are anticipated for a single procedure. This is especially important for the larger patient population.
- 9.
Stand as a far as possible from the point where the x-ray beam enters the patient.
Protection From Radiation of C-Arm Equipment When in Operation
The three tenets of radiation protection are:
- 1.
Time. Reduce the amount of time you are exposed to radiation, and you reduce your total exposure (again, use pulsed rate fluoroscopy, use last image hold devices).
- 2.
Distance. Maintain a safe distance away from the radiation source. Recall how the “inverse-square” law greatly influences the intensity of radiation with changing distance. Increase your distance away from the x-ray beam and patient whenever possible.
- 3.
Shielding. When working with an x-ray source, you must wear an appropriate lead-equivalent gown of 0.35 mm lead equivalency or greater (0.25 mm lead equivalency with a mini C-arm device). To avoid cracking the shielding inside the protective garment, store these on suitable racks or laid out unfolded on a table until next use ( Fig. 13A-21 ).
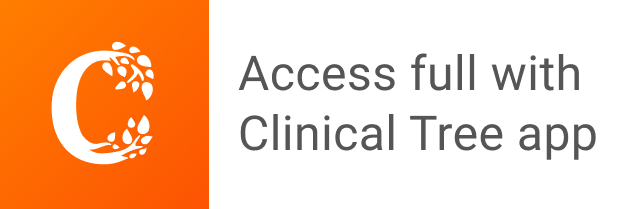