Advanced imaging techniques are promising tools to assist in the early diagnosis and monitoring of therapy in various rheumatic diseases. As there is now increasing emphasis on diagnosing inflammatory rheumatic disease in the pre-clinical stages, so that treatment may be instituted early and ideally prevent irreversible tissue damage, highly sensitive techniques are needed to detect subclinical inflammation. Moreover, there is an increasing need to develop individualised treatment protocols at reasonable cost and with optimal therapeutic effect. Tools are required that can image the therapeutic target and sensitively trace changes in disease activity.
Nuclear imaging techniques have the potential to fulfil these clinical needs. Positron emission tomography is emerging as an important modality as it provides highly sensitive, quantitative imaging at a molecular level, to reveal the important pathophysiological processes underlying inflammation. This chapter provides an overview of currently available nuclear imaging techniques, including recent technical developments, and discusses their role in the diagnosis and monitoring of rheumatic disease.
Introduction
Timely diagnosis and early effective treatment can improve the outcome of various inflammatory rheumatic diseases . To enable early diagnosis and develop individualised therapeutic protocols, sensitive monitoring tools such as advanced imaging techniques are needed. Promising results have already been obtained with anatomical imaging modalities including magnetic resonance imaging (MRI) and ultrasound (US), with highly sensitive detection of synovitis and bone marrow oedema in inflammatory arthropathies and vascular thickening in systemic vasculitides . Each technique, however, has drawbacks and limitations, as MRI usually produces images from only a limited field of view and US is operator-dependent and laborious if multiple sites need to be examined. In addition, both techniques visualise indirect signs of inflammation, in terms of either increased tissue water content and/or contrast enhancement as a result of hyperperfusion at the site of inflammation. Since the diagnosis of rheumatic disease and the assessment of disease activity are moving towards the subclinical end of the disease spectrum, nuclear imaging techniques may assume increasing importance. These techniques rely on visualisation of pathophysiological changes in tissues. In the 60s, nuclear imaging, that is, planar bone scintigraphy was introduced for the imaging of arthritis. This was followed by the development of more specific radiolabelled agents (such as radiolabelled immunoglobulin) in the 80s, then the introduction of single photon emission computed tomography (SPECT) and subsequently, in the 90s, positron emission tomography (PET). In the last few years, hybrid techniques as PET-computed tomography (CT) and PET-MRI also became available.
In the first part of the chapter (section Nuclear medicine imaging techniques ), the different nuclear imaging techniques are explained, including strengths and weaknesses relevant to their use in rheumatic disease. Subsequently, the potential role of nuclear imaging in inflammatory rheumatic diseases is discussed, focussing on rheumatoid arthritis (RA), ankylosing spondylitis (AS) and large vessel vasculitis (LVV) respectively, in sections Nuclear imaging of RA , Nuclear imaging of AS and Nuclear imaging of LVV . Most of the summarised data are derived from feasibility studies in clinically active disease. However, nuclear imaging techniques are most likely to find their place as tools for early diagnosis, in particular in the preclinical phase, and in monitoring of therapy. These data, if available, are also included in the relevant sections.
Nuclear medicine imaging techniques
General characteristics
Nuclear medicine imaging is based on the detection of gamma rays (sometimes also called photons). Some unstable isotopes emit gamma rays when they decay to the ground state. Based on details of the composition of the unstable isotope, this decay can be accomplished by either the direct emission of a single gamma ray (as is the case for 99mTc) or the indirect emission of two gamma rays (as is the case for 18F, which is a positron emitter). A combination of a biologically active molecule and such an unstable isotope is called a radiopharmaceutical. It is important to note that although the number of radioisotopes having properties that make them useful for imaging is limited, the potential number of radiopharmaceuticals is very large. This will be illustrated further on in this chapter.
To begin the imaging procedure, the patient is injected with a radiopharmaceutical and, as a result, gamma ray emissions originating from within the patient can be detected by an external detector. The newer techniques, such as SPECT and PET, both carry the word ‘emission’ in their name, bearing evidence to the fact that the gamma rays that are being detected originate from within the body. This is in stark contrast to the X-rays of a CT scan, where the source and the detector are outside the patient and the patient is only subjected to radiation as long as the X-ray source is turned on. The ‘computed tomography’ part of SPECT and PET refers to their ability to produce three-dimensional (3D) images of the region of interest within the patient. In order to do so, the gamma rays emitted from the patient need to be measured for a number of projections (a projection refers to gamma rays travelling with a known and fixed orientation with respect to the origin of the field of view of the camera). This contrasts with planar scintigraphy, which only allows for 2D images to be acquired (single projections).
Until recently, there was hardly any exception to the rule that all measuring equipment was based on the combination of a scintillation crystal and a photomultiplier tube. In the scintillator, a gamma ray is converted into optical photons. The photomultiplier tube converts optical photons into photo-electrons, which are subsequently multiplied to yield a signal that is proportional to the energy of the incoming gamma ray and large enough to be processed by standard electronics. Introduced in the 1950s, this measurement technique is still ubiquitous in every clinical nuclear medicine department. The problematic dependence of the multiplication efficiency of photomultiplier tubes on magnetic fields (they even need to be shielded for the earth’s magnetic field) has not been a problem until the recent introduction of PET-MRI, which has necessitated finding an alternative for this measurement technique. A number of excellent books exist that discuss the physics and some other general aspects of nuclear medicine imaging . The reader is referred to those for a more in-depth discussion of the topics briefly mentioned above.
Single photon imaging (planar scintigraphy and SPECT)
Single photon imaging was the first clinically relevant imaging technique. In order to acquire a single projection of gamma ray data, the detection of gamma rays needs to be limited to those with a well-known orientation in space. In single photon imaging, this is accomplished by placing a collimator in front of the scintillation crystal. A collimator ensures that incoming gamma rays are only detected when they travel perpendicular to the detector. This works well, but comes with a clear disadvantage: they allow for only a few percent of the emission gamma rays travelling in the direction of the detector panel to be detected (those not perpendicularly incident will be stopped in the collimator). Typically, single photon imaging is performed with a camera that consists of one or more panel detectors.
Where a stationary panel is used, the technique is called planar scintigraphy. Since only one projection is obtained, only planar images result. Usually, an anterior and a posterior image together form the basis for the diagnostic process. Where one or more rotating panels are used, the technique is called SPECT. By rotating the panels, more than one projection can be acquired, allowing for full tomographic reconstruction of the emission data and therefore producing a 3D image.
There is another dimension that is sometimes of interest – time. It is sometimes helpful to monitor the presence of activity over time in a certain region. With scintigraphy, such an approach is possible, assuming that the panel detector covers the complete region of interest. SPECT approaches require multiple projections and therefore the detection panels need to rotate, thereby limiting the temporal resolution.
Positron emission tomography (PET, PET-CT and PET-MRI)
From the 1970s, a more sensitive technique was introduced in nuclear medicine, based on positron emitting radioisotopes. A positron loses its energy due to collisions with atoms and electrons and, after almost all energy is lost, it annihilates with an electron to form two gamma rays. These two gamma rays will be travelling back to back from the point of annihilation. This intrinsic property of positron emitters (the fact that two gamma rays with a fixed 180° orientation will result from their annihilation) provides a number of advantages over SPECT:
- i)
Detection of both of these two photons will be enough to determine their orientation: it will be along the path connecting the two interaction points. Since a collimator is not needed anymore, the detection sensitivity is ∼100 times higher (nanomolar range) than in SPECT.
- ii)
PET detectors are usually circular, allowing for the simultaneous acquisition of multiple projections. 4D images (the 3D radioactivity distribution as a function of time) can be acquired with a time resolution in the order of 10 s.
- iii)
Due to the physics of the interaction of gamma rays with matter, the two gamma rays resulting from annihilation of a positron can be corrected for the attenuation and scatter probability during their travel from within the patient to the detector. This is not the case for a single gamma ray emission.
Coincidence detection is therefore the outstanding requirement for PET imaging. Current electronics limit the acceptable time difference between two events to less than ∼5 ns, but this is still relatively long and therefore corrections to the measured number of coincidences must be made. These corrections include normalisation for detector (or line of response), efficiency, scatter and attenuation correction. Contrary to the situation in single photon emission, attenuation and scatter correction can be carried out accurately in PET, leading to the claim that PET imaging is quantitative. Knowledge of the attenuation properties of the object being measured is necessary. Until the 1990s, attenuation was measured with radionuclides, which were produced by an external gamma ray source. However, the realisation that the attenuation properties could also be measured with X-ray CT led to the introduction of PET-CT systems. Since the CT image not only defined the attenuation properties, but also allowed for spatial localisation of the PET signal, it did not take long before it became virtually impossible to buy a stand-alone PET scanner. Due to the limited soft-tissue contrast present in CT images as well as the radiation dose associated with certain applications (∼3 mSv for a whole-body low-dose CT scan needed for attenuation correction), there has been a recent drive towards the development of PET-MRI scanners.
Strengths and weaknesses
Table 1 presents a rather coarse overview of strengths and weaknesses of the various techniques that were briefly introduced above. In the text below the table the various issues are briefly discussed.
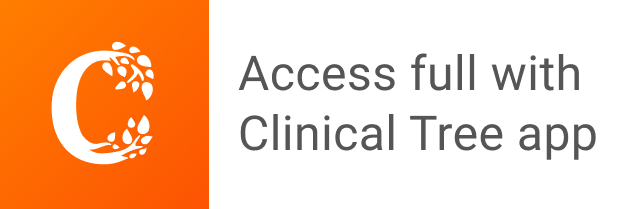