Abstract
Chronic pain is an important public health problem, and there is a need to understand the mechanisms that lead to pain chronification. From a neurobiological perspective, the mechanisms contributing to the transition from acute to subacute and chronic pain are heterogeneous and are thought to take place at various levels of the peripheral and central nervous system. In the past decade, brain imaging studies have shed light on neural correlates of pain perception and pain modulation, but they have also begun to disentangle neural mechanisms that underlie chronic pain.
This review summarizes important and recent findings in pain research using magnetic resonance tomography. Especially new developments in functional, structural and neurochemical imaging such as resting-state connectivity and γ-aminobutyric acid (GABA) spectroscopy, which have advanced our understanding of chronic pain and which can potentially be integrated in clinical practice, will be discussed.
Chronic pain: prevalence and concepts
Chronic pain is an important public health problem. The prevalence of chronic pain in Western, industrialized countries is estimated to be between 15% and 20% of the adult population . As such, there is a need to better understand the mechanisms that lead to pain chronification.
From a neurobiological perspective, the mechanisms contributing to the transition from acute to subacute and chronic pain are heterogeneous and are thought to occur both within the peripheral nervous system and at various levels of the central nervous system (CNS). The role of the brain in chronic pain states remains to be fully elucidated; however, it has now been widely accepted that chronic pain cannot be thought of as an endless string of nociceptive inputs to an otherwise normally functioning brain, but that neuroplastic remodelling occurs on various levels of the nervous system ranging from synaptic plasticity to reorganization of large-scale neural networks, which can lead to the maintenance of pain, even in the absence of the original nociceptive input. With respect to the underlying pathophysiology, it is worth noting that there has been a substantial paradigm shift in thinking about the neurobiology of chronic pain. Until recently, chronic pain had been largely viewed as ‘acute pain that is lasting too long’, that is, that chronic pain results from an endless string of ongoing peripheral nociceptive or neuropathic inputs to an otherwise healthy/normal brain ; in other words, a patient experiences chronic pain because of a prolonged/repetitive nociceptive input originating from (sensitized) nociceptors (as in osteoarthritis), or irritated/damaged nerve fibres (as in demyelinating polyneuropathy, or nerve compression), which leads to a chronic stimulation of the central pain system, thus sustaining pain. However, this concept has been fundamentally challenged. There is now a general agreement that the CNS plays a prominent role in many chronic pain states due to the ‘centralization’ of pain. Some of the findings that strongly support this paradigm shift are outlined subsequently.
In addition to what are (or thought to be) obvious reasons for chronic pain (e.g., chronic inflammatory diseases, polyneuropathies, etc.), there are many patients for whom no adequate structural correlates for their pain can be found. This group of conditions includes chronic pain states such as chronic tension-type headache, temporomandibular disorder, irritable bowel syndrome (IBS), unspecific chronic low back pain, fibromyalgia and chronic pelvic pain (CPP) , and this represents the most common causes of chronic pain until patients reach advanced age and develop osteoarthritis.
Even in conditions where a pain generator in terms of a nociceptive source can be identified, the degree of measurable change/damage (e.g., in terms of joint narrowing in osteoarthritis) correlates only weakly with the degree of pain experienced . Similarly, most individuals with diabetic neuropathy feel no pain, or even have decreased sensation, whereas others with the same peripheral ‘lesion’ suffer from severe pain or allodynia. These findings imply that cortical processing of the nociceptive input, as well as interindividual variability in antinociceptive mechanisms, plays a crucial role in the transition from nociception to conscious pain perception. Finally, in addition to pain per se, patients with chronic pain often suffer from additional symptoms, including depressive episodes, anxiety and chronic fatigue . These co-morbidities add significantly to the degree of suffering in patients with chronic pain and are most likely caused by central mechanisms.
Brain imaging, as a tool to assess brain function, structure and chemistry, in this regard has had a great influence on our understanding of the neural correlates of pain perception and pain modulation, and it holds promise in further unravelling central mechanisms that contribute to pain chronification.
Neuroimaging in experimental pain conditions
In the past two decades, a variety of functional brain imaging techniques in human subjects, including functional magnetic resonance imaging (fMRI), positron emission tomography (PET), electroencephalography (EEG) and magnetoencephalography (MEG), as well as animal studies have shed light on the way in which the brain reacts to nociceptive stimuli (acute pain), thereby helping to establish the concept of the so-called ‘pain system’ . The most influential technique has been fMRI due to its fairly high temporal resolution and the fact that no contrast agents or radioactive substances are required to image neural activation. The current review focuses on MRI findings, while acknowledging that other methods such as single photon emission computed tomography and PET have also significantly contributed to the field, both with respect to brain activation (functional imaging) as well as in the context of metabolic and pharmacological imaging .
fMRI is based on measuring and analysing the so-called BOLD (blood oxygen level dependence) effect. An increase in neural activity leads to a haemodynamic response, associated with an increase in regional blood flow and volume resulting in an increase of the oxyhaemoglobin–deoxyhaemoglobin ratio, which in turn leads to a reduction of local magnetic inhomogeneity. A decrease in magnetic inhomogeneity is in turn associated with slower dephasing of protons, which causes an increase in the signal in T 2 *-weighted images (in most cases, echoplanar imaging (EPI) is used). Activation maps are then created by fitting original data to a model and assessing the quality of the fit. The model is set up by the investigator on the basis of the ‘on’ condition (when an experimental pain stimulus is presented) and the ‘off’ condition (rest or non-painful stimulus is presented), and a standardized haemodynamic response function, predicting changes in the BOLD signal during the time series. As such, the BOLD signal is only an indirect marker of neural activity, and how excitatory and inhibitory neural activity adds up to influence the BOLD signal remains to be fully elucidated .
A fairly new technique, which has only recently been applied in the pain field, is arterial spin labelling (ASL) . ASL is an MRI technique that uses water in arterial blood as a freely diffusible tracer to measure perfusion noninvasively. This approach allows for the quantification of regional cerebral blood flow (rCBF) as a surrogate marker of neural activity, whereas the BOLD signal reflects a composite effect of changes in blood flow, vascular volume and oxygen metabolism.
Activation sites across studies vary to some degree, depending on experimental paradigm and pain stimulus (i.e., heat, cold pressure, electric shock, ischemia, etc.). However, the main components of this network are the primary and secondary somatosensory cortex (SI and SII), the insular cortex (IC), the anterior cingulate cortex (ACC), the prefrontal cortices (PFC) and the thalamus . Accordingly, the pain system may be said to involve somatosensory, limbic and associative brain structures. The two brain sites that most consistently show activation in response to acute pain stimuli are the ACC and the IC . These regions are thought of as multisensory integration sites, and they have been implicated in various aspects of pain experience and assessment , such as the anticipation , affective processing of pain and antinociception . Motor and premotor areas (supplementary motor area, SMA) and the dorsolateral prefrontal cortex (DLPFC) have also been shown to be activated by pain stimuli, but these activations are less reliable, and it is unclear whether they are directly related to pain perception or represent epiphenomena, such as pain-evoked movements, movement suppression and/or top down modulation, respectively.
This network of concomitantly activated brain areas, in response to a nociceptive stimulus, is often referred to as the ‘pain matrix’, and it has been thought to be specific to pain perception, decoding distinct aspects of pain such as pain intensity and unpleasantness. However, this notion has been challenged by various empirical findings, which indicate that, for example, the pain intensity can be dissociated from the magnitude of the neural response within the pain matrix and that other, non-painful stimuli can also activate cortical and subcortical areas in the same spatial configuration of the pain matrix. A counterproposal suggests that the system more generally reacts to salient sensory events, occurring to the body or in the surroundings and being potentially harmful to the body’s integrity .
In the context of chronic pain, it is important to acknowledge that ‘new’ brain areas, not part of the traditional ‘experimental’ pain system, such as the nucleus accumbens (NAc), the hippocampus, frontopolar regions and the parietal lobe have been found to correlate with specific aspects of pain perception. Against this background, neural circuitry involved in acute pain perception and chronic pain suffering at least partially dissociate (for more details, see the following sections).
Neuroimaging in experimental pain conditions
In the past two decades, a variety of functional brain imaging techniques in human subjects, including functional magnetic resonance imaging (fMRI), positron emission tomography (PET), electroencephalography (EEG) and magnetoencephalography (MEG), as well as animal studies have shed light on the way in which the brain reacts to nociceptive stimuli (acute pain), thereby helping to establish the concept of the so-called ‘pain system’ . The most influential technique has been fMRI due to its fairly high temporal resolution and the fact that no contrast agents or radioactive substances are required to image neural activation. The current review focuses on MRI findings, while acknowledging that other methods such as single photon emission computed tomography and PET have also significantly contributed to the field, both with respect to brain activation (functional imaging) as well as in the context of metabolic and pharmacological imaging .
fMRI is based on measuring and analysing the so-called BOLD (blood oxygen level dependence) effect. An increase in neural activity leads to a haemodynamic response, associated with an increase in regional blood flow and volume resulting in an increase of the oxyhaemoglobin–deoxyhaemoglobin ratio, which in turn leads to a reduction of local magnetic inhomogeneity. A decrease in magnetic inhomogeneity is in turn associated with slower dephasing of protons, which causes an increase in the signal in T 2 *-weighted images (in most cases, echoplanar imaging (EPI) is used). Activation maps are then created by fitting original data to a model and assessing the quality of the fit. The model is set up by the investigator on the basis of the ‘on’ condition (when an experimental pain stimulus is presented) and the ‘off’ condition (rest or non-painful stimulus is presented), and a standardized haemodynamic response function, predicting changes in the BOLD signal during the time series. As such, the BOLD signal is only an indirect marker of neural activity, and how excitatory and inhibitory neural activity adds up to influence the BOLD signal remains to be fully elucidated .
A fairly new technique, which has only recently been applied in the pain field, is arterial spin labelling (ASL) . ASL is an MRI technique that uses water in arterial blood as a freely diffusible tracer to measure perfusion noninvasively. This approach allows for the quantification of regional cerebral blood flow (rCBF) as a surrogate marker of neural activity, whereas the BOLD signal reflects a composite effect of changes in blood flow, vascular volume and oxygen metabolism.
Activation sites across studies vary to some degree, depending on experimental paradigm and pain stimulus (i.e., heat, cold pressure, electric shock, ischemia, etc.). However, the main components of this network are the primary and secondary somatosensory cortex (SI and SII), the insular cortex (IC), the anterior cingulate cortex (ACC), the prefrontal cortices (PFC) and the thalamus . Accordingly, the pain system may be said to involve somatosensory, limbic and associative brain structures. The two brain sites that most consistently show activation in response to acute pain stimuli are the ACC and the IC . These regions are thought of as multisensory integration sites, and they have been implicated in various aspects of pain experience and assessment , such as the anticipation , affective processing of pain and antinociception . Motor and premotor areas (supplementary motor area, SMA) and the dorsolateral prefrontal cortex (DLPFC) have also been shown to be activated by pain stimuli, but these activations are less reliable, and it is unclear whether they are directly related to pain perception or represent epiphenomena, such as pain-evoked movements, movement suppression and/or top down modulation, respectively.
This network of concomitantly activated brain areas, in response to a nociceptive stimulus, is often referred to as the ‘pain matrix’, and it has been thought to be specific to pain perception, decoding distinct aspects of pain such as pain intensity and unpleasantness. However, this notion has been challenged by various empirical findings, which indicate that, for example, the pain intensity can be dissociated from the magnitude of the neural response within the pain matrix and that other, non-painful stimuli can also activate cortical and subcortical areas in the same spatial configuration of the pain matrix. A counterproposal suggests that the system more generally reacts to salient sensory events, occurring to the body or in the surroundings and being potentially harmful to the body’s integrity .
In the context of chronic pain, it is important to acknowledge that ‘new’ brain areas, not part of the traditional ‘experimental’ pain system, such as the nucleus accumbens (NAc), the hippocampus, frontopolar regions and the parietal lobe have been found to correlate with specific aspects of pain perception. Against this background, neural circuitry involved in acute pain perception and chronic pain suffering at least partially dissociate (for more details, see the following sections).
Neuroimaging in chronic pain conditions: fMRI
As compared to experimental pain with clear-cut ‘on’ and ‘off’ conditions that can be applied reproducibly in the scanner environment, the delineation of the neural correlates of chronic, spontaneous pain is more challenging. Many fMRI studies, investigating patients with chronic pain, have examined brain responses to an experimental pain stimulus. In certain circumstances, this might be a valid approach, for example, when investigating patients with allodynia, that is, a pain sensation triggered by an external stimulus; however, there is no compelling evidence that examining brain activity in response to experimental pain stimuli can predict the pattern of brain activity in chronic spontaneous pain.
In recent years, new imaging techniques have evolved to delineate new aspects of chronic pain perception and neural correlates thereof. One such approach is the analysis of resting-state activity and connectivity. Using an elegant approach, Baliki et al. investigated patients with chronic low back pain and demonstrated that clinical pain, while patients were lying in the scanner, correlated with neural activity as delineated by the BOLD signal in the medial PFC and partially in the ICs . With regard to resting-state activity, that is, spontaneous BOLD fluctuations and the power in the high frequency range (0.12–0.20 Hz), the same group could identify the medial PFC to display increased BOLD oscillation in patients with back pain as compared to healthy controls . Using ASL, to measure rCBF, Wasan et al. could show that worsening of ongoing back pain was associated with increased blow flow in regions inside and outside the ‘classical’ pain system, for example, the somatosensory, prefrontal and insular cortices, but also the superior parietal lobule, which is part of the dorsal attention network .
As opposed to regional activity, functional connectivity (fc) has been operationally defined to refer to temporal correlations across cortical regions. Specifically, ‘resting-state’ fc refers to brain areas that have a strong temporally correlated (<0.2-Hz) activity in a non-task state. It is thought that these low-frequency fluctuations are functionally relevant indices of connectivity between brain regions subserving similar or related brain functions Fig. 1 .
So far, fc has been investigated in several chronic pain states, mainly focusing on the fc of the salience network as well as the default mode network (DMN) . Investigating the fc of the DMN in patients with diabetic neuropathic pain, Cauda and colleagues reported an increased connectivity between the DMN and the dorsal ACC and the SI/MI cortex bilaterally (among others) and a decreased connectivity between the DMN and the DLPFC, frontopolar cortex, the IC and the thalamus bilaterally (among others) . Likewise, investigating the fc of the DMN in patients with fibromyalgia and healthy controls, Napadow et al. showed that patients with fibromyalgia had greater connectivity between the DMN and the IC . Interestingly, in a subsequent study, investigating analgesic mechanisms of pregabalin in this patient group, reductions in clinical pain were associated with reductions in fc between the DMN and the posterior IC, corroborating that the interaction of these two regions might play a specific role in chronic pain . Critically, it must be noted that for intervention studies the change of neural activity or connectivity may not be identical with the primary site of action (of the drug or the nonpharmacological treatment), but it might reflect reduced pain perception following an intervention effect taking place at some other location, for example, the spinal cord.
Interestingly, other brain regions and their connectivity have also been identified to correlate with pain and/or pain improvement, such as the PAG, the DLFPC and the NAc . Although both the PAG and the DLPFC have clear implications in pain perception and modulation, the NAc has a well-established role in reward processing, but less so in pain processing. However, it has been suggested that the NAc might specifically play a role in coding adversity of pain, linking pain sensation to emotion. Only recently has it been shown that NAc connectivity is altered in patients with chronic low back pain and might even predict the transition from subacute to chronic pain . As such, it becomes increasingly evident that the brain networks involved in chronic clinical pain conditions at least partially differ from those seen in acute pain perception (in healthy subjects) inasmuch as chronic pain involves brain regions critical for cognitive and emotional processing/assessment .
Neuroimaging in chronic pain conditions: structural brain imaging
In most cases, structural brain imaging is based on high-resolution T1-weighted images that are particularly suited to delineate the border between grey and white matter and that can be used to assess global measures, such as whole brain volume, grey and white matter volume, as well as regional features, including cortical thickness and voxel-based parameters, for example, grey or white matter values, interpreted as regional grey/white matter density or volume.
Structural alterations of the brain in people with chronic pain have now been reported in a wide range of chronic pain conditions such as headaches and facial pains , chronic widespread pain , back pain , chronic visceral pain as well as chronic neuropathic pain . The first studies applying VBM to detect alterations in regional brain morphology in patients with chronic pain reported a decrease in grey matter in the thalamus and DLPFC in patients with chronic back pain, and a decrease in grey matter in the ACC, mid-cingulate and posterior cingulate cortex, the IC bilaterally, the mesial temporal lobe and the brain stem in patients suffering from chronic tension-type headache. Since then, a number of studies have identified grey matter decreases in various pain syndromes, mostly in structures related to pain perception and modulation (e.g., DLPFC) and antinociception (e.g., pregenual/subgenual ACC ). Some studies also reported increases in grey matter in the basal ganglia (globus pallidus/putamen) in patients with chronic back pain , fibromyalgia , vulvodynia and temporomandibular disorder . A recently published meta-analysis based on 23 VBM studies investigated various chronic pain conditions and described reductions in grey matter volume/density in several areas, including the IC bilaterally, the ACC, the supplementary motor area, the thalamus and the PFC (the left inferior frontal gyrus and right medial frontal gyrus). The one region with higher grey matter volume was identified in the right medial temporal lobe.
In a number of studies, cortical thickness analyses have revealed decreases in cortical thickness, such as in the cingulate cortex of patients with trigeminal neuropathic pain , fibromyalgia , and patients with IBS . On the other hand, increases in cortical thickness have been reported in the somatosensory cortices of patients with migraine , those with chronic low back pain , and female patients suffering from an IBS . Overall, the alterations seen in chronic pain syndromes seem to fall into one of two patterns. Most of the studies report a decrease in grey matter density/volume or a decrease in cortical thickness in structures known to be part of the salience network or regions involved in antinociception, or cognitive pain modulation (i.e., the dorsal ACC and MCC, the IC, the pregenual ACC and the DLPFC). Increases in regional grey matter, on the other hand, have been described in the basal ganglia , whereas cortical thickness increases were mainly found in the primary sensory cortex .
New approaches such as T1 and T2* mapping magnetization transfer ratios (MTRs) have also recently been applied to pain conditions. Granziera et al. reported that migraineurs (with aura) displayed a significantly shorter T1 relaxation time in the thalamus as compared to migraineurs without aura and healthy controls . In addition, MTR was higher and T2* relaxation time was significantly shorter (in the thalamus) in migraineurs with aura as compared to those without aura. The authors suggest that these alterations might be due to increased myelin content/cellularity and iron deposition, which in turn is possibly related to abnormal cortical excitability control.
The neurobiological correlates of changes in regional brain morphology associated with chronic pain, in terms of tissue composition, are still not fully understood. Grey matter decreases are likely to reflect tissue shrinkage affecting neural tissue (neurons and glia), vasculature and extracellular volume. In the light of spectroscopy findings (see below), it has been suggested that grey matter changes (decreases) might result from neurodegenerative processes that affect the size or number of neurons and/or neuroglia . It has been suggested that some chronic pain conditions are associated with decreased GABA (γ-aminobutyric acid) levels (measured in supraspinal structures, e.g., IC) . If neuronal loss preferentially affected inhibitory interneurons, it is conceivable that increased (spontaneous) activity in areas known to be involved in pain processing might influence subsequent pain perception and contribute to spontaneous pain, at the same time leading to grey matter decreases as revealed by structural brain imaging . Astrocytes, as another subtype of neuroglia, are critically involved in potassium homeostasis and glutamate reuptake, thereby having a direct impact on neuronal activity and metabolism . However, the notion of a neurodegenerative process has been challenged by several VBM studies that describe a reversibility of grey matter decreases either spontaneously or after surgical intervention treating a peripheral, nociceptive source .
Diffusion tensor imaging (DTI), which provides measures of directed water diffusion, such as fractional anisotropy (FA) and mean diffusivity (MD), is regarded as a marker of white matter integrity. DTI can also be used to perform tractography, that is, the three-dimensional (3d) modelling of neural tracts, which can then be applied to assess the connectedness of remote brain regions, therefore providing a marker of structural connectivity. In one of the first studies applying DTI and tractography, Gehaet al. reported that a patient population suffering from a complex regional pain syndrome displayed a decrease in FA in the left cingulum-callosal bundle, as well as altered white matter connectivity between the ventromedial PFC and the insula (increased connectivity) and between the ventromedial PFC and the basal ganglia (decreased connectivity) . Using tractography, higher degrees of structural connectivity between the thalamus, the PFC and the ACC, as well as decreased connectivity between the thalamus and the basal ganglia, have also been reported in patients with IBS as compared with a control group . Another study investigating patients with temporomandibular disorder identified the corpus callosum to have higher connectivity with the frontal pole, and lower connectivity to the DLPFC . These studies underline the importance of considering alterations in structural connectivity, especially of the pain system and salience network when describing altered brain structure in patients with chronic pain.
Neuroimaging in chronic pain conditions: spectroscopy
Although fMRI and structural magnetic resonance imaging (sMRI) are based on radiofrequencies of protons within water molecules, proton magnetic resonance spectroscopy (H-MRS), as applied in most human imaging studies, is used to measure radiofrequencies of protons bound to carbon atoms, that is, organic compounds. Due to specific signatures of the chemical shift, this method allows the quantification of certain metabolites/compounds, such as N-acetyl-aspartate (NAA), choline, creatine, myoinositol and lactate, which are thought to mirror certain aspects of neuronal or astrocyte integrity/metabolism. NAA, for example, is thought of as a marker of neuronal density or cell viability, whereas creatine reflects adenosine triphosphate metabolism.
Grachev et al. described lower NAA levels within the DLPFC of patients with chronic back pain as compared with healthy controls . Lower NAA levels have also been reported in the thalamus of patients with neuropathic pain included . These decreases in NAA might possibly reflect a neuronal loss, indicating a neurodegenerative process to be associated with chronic pain.
More recently, H-MRS has been used to measure local concentrations of neurotransmitters, for example, glutamate (where GLX is a composite measure of glutamate and glutamine) . Technical advances in H-MRS, the development of the so-called editing sequences, for example, MEGA-PRESS spectroscopy, have also enabled the quantification of GABA . In the context of chronic pain, Harris et al. could show that patients with fibromyalgia had increased glutamate levels in the IC and that changes in posterior insular glutamate (and GLX) levels correlated with changes in both experimental pain thresholds and clinical pain . Other groups also reported on elevated GLX levels in various brain sites in patients with fibromyalgia, such as the amygdala and the posterior cingulate cortex . Decreased GLX levels have also been found in other chronic pain syndromes. Niddam et al., for example, reported decreased GLx levels in the hippocampus of IBS patients, indicating that altered transmitter concentration might be region and/or disease specific . GABA has been investigated less frequently. Decreased GABA levels have been reported to be present in the IC of patients with fibromyalgia as well as patients with diabetic neuropathic pain . In a multimodal imaging study, using H-MRS and ASL, Gustin et al. could demonstrate that patients with neuropathic pain following spinal cord injury display lower levels of thalamic NAA, GABA and regional blood flow (in the thalamus) as compared to healthy controls and patients with spinal cord injury but no pain . This and other studies support the notion that neuropathic pain is associated with CNS reorganization, specifically within the thalamus, comprising functional as well as neurochemical mechanisms.
The quantification of brain metabolites as well as neurotransmitters is of high interest, as it can provide indirect evidence of local neural activity and/or excitability, possibly even being a predictor for therapy response . The relationship between local metabolite/neurotransmitter concentration and brain function, for example, the BOLD signal, and brain structure, for example, grey matter density, remains to be investigated further. Furthermore, whether altered neurotransmitter concentrations in patients with chronic pain reflect a global- (whole brain) or region-specific phenomenon, for example, confined to regions in the pain system needs to be investigated in more depth.
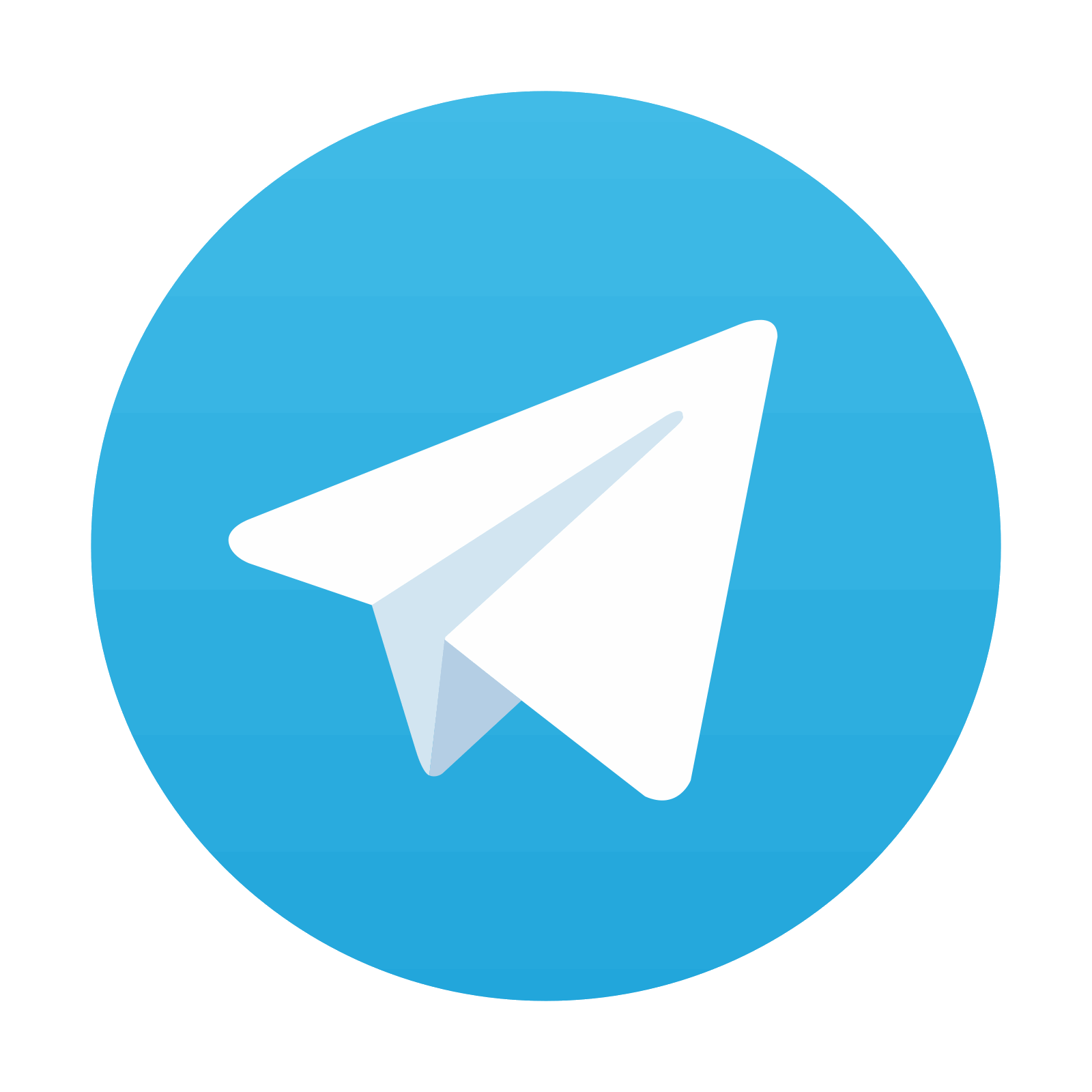
Stay updated, free articles. Join our Telegram channel
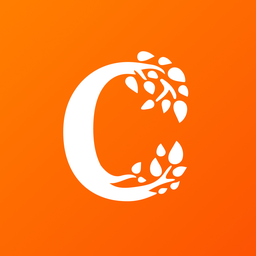
Full access? Get Clinical Tree
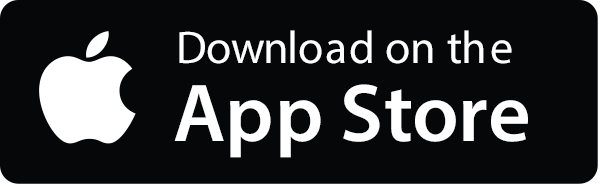
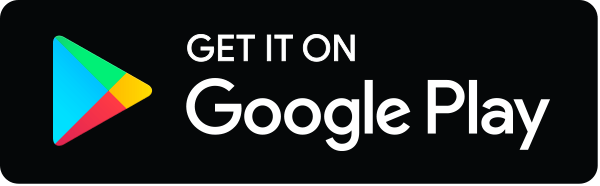