The nervous system is unique in its ability to accept and interpret various stimuli and develop a response to that stimuli, whether action based or emotional. In addition to seemingly voluntary outputs, it works to involuntarily maintain homeostasis throughout the body. The highest concentration of neurons, the basic unit of our nervous system, is within the brain and numbers in the tens of billions.1 When regarding the nervous system as a whole, the total number of neurons has been hypothesized to exceed 100 billion. Though knowledge of the architecture of our nervous system is quite vast, novel research is constantly pushing the boundaries of our understanding and providing new insights into this incredibly intricate system. Through the context of this chapter we will touch upon aspects of the central and peripheral nervous system, including its anatomy and physiology. In addition to this we will outline ways to interpret the health of a patient’s nervous system through physical examination and diagnostic tools. This text is meant to serve as a primer of information to create a foundation. At times details may be purposefully foregone as they will be discussed in later chapters with detail.
The nervous system as a whole is divided into the central nervous system (CNS) and the peripheral nervous system (PNS). The CNS consists of the brain and the spinal cord, and the peripheral nervous system consists of the individual nerves with nerve fibers that either exit the spinal cord or feed into it from the periphery. The movement of information toward the CNS and ascending to the brain is considered afferent signaling, whereas information descending down the spinal cord and exiting the CNS structures to end organs, muscles, and glands is considered efferent signaling. The PNS is then further divided into the somatic and autonomic nervous systems. The somatic nervous system consists of peripheral nerves responsible for motor control of body movements as well as sensory information. The autonomic nervous system is responsible for unconscious bodily functions and homeostasis through nerve innervations to viscera, smooth muscle, and glandular structures. The autonomic nervous system is even further divided into the sympathetic and parasympathetic nervous system, each with specific functions (Figs. 3–1 and 3–2).
Critical to our understanding of the nervous system is the anatomy of a nerve cell or neuron. A neuron consists of a cell body with receptive extensions known as dendrites, a long process known as an axon, and a presynaptic terminal that makes contact with end organs, glands, vessels, muscles, or other neurons (Fig. 3–3). Most nerves are multipolar, meaning that they characteristically have one long axonal extension from their cell body and a vast array of dendritic branches. An exception exists within the sensory nervous system, which typically consists of bipolar or pseudo-unipolar nerve cells (Fig. 3–4). A stimulus is directly received at the dendritic extension or the cell body and is then transmitted along the length of the axon toward the synapse. The synapse is the junction point between two neurons where nerve signals are passed forward. The branch architecture of the dendrite, as well as the number of receptors and voltage-gated ion channels, determine how the nerve cell integrates information from a stimulus. All neurons maintain a voltage gradient across their membrane that, when stimulated, helps propagate a signal along the length of the axon. The branch and receptor architecture of the dendrite, as well as characteristics of the axon and presynaptic terminal, play an important role in defining the nerve type.
Three general nerve types include motor, sensory, and autonomic nerves. Motor nerves conduct information in an efferent manner toward voluntary skeletal muscles and smooth muscles of internal organs and glands. These neural transmissions are usually generated from a central source. In contrast, sensory nerves are primarily afferent in nature and transmit information from a source external to the nervous system. For example, temperature sensation is received by sensory receptors and travels in an afferent manner along a peripheral nerve to the central nervous system. Autonomic nerves tend to be mixed efferent-afferent and are utilized for regulation of bodily functions and homeostasis.
Two important characteristics that determine the speed at which a signal is propagated are the diameter of the nerve axon and its insulation. As a rule, larger-diameter axons conduct action potentials at a faster conduction velocity than smaller-diameter axons. Studies have even demonstrated that a reduction in cross-sectional area of nerves in certain neuropathic disease processes correlate to a reduction in nerve conduction velocity.2 Myelin, a substance composed of protein and phospholipids, forms a sheath around the axon providing insulation to create a boundary for the electric charge. Myelinated axons are white in appearance and provide the color contrast of “white matter” from “gray matter.” The myelin sheath prevents electric charges from leaving the axon, and the degree of myelination is positively correlated with the velocity at which a signal can be conducted through the axon. Hence, the higher the myelination, the higher the conduction velocity. Gaps in the myelin sheath along the axon are called nodes of Ranvier, and only at these gaps can an abundance of voltage-gated channels be found (Fig. 3–5). These nodes are the only locations for ion exchange along the length of the axon and thus the only location for a substantial electrochemical gradient shift. This method by which action potentials propagate along an axon from node to node is called salutatory conduction. Alterations in myelin and nodal structure have been identified in sensory neuropathic disease processes like diabetic neuropathy3 and are correlated with a decrease in nerve conduction. The velocity at which action potentials propagate is directly related to the myelination, diameter of the neuron, and nodal architecture.
Figure 3–5
Motor neuron with a myelinated axon. A motor neuron is composed of a cell body (soma) with a nucleus, several processes called dendrites, and a long fibrous axon that originates from the axon hillock. The first portion of the axon is called the initial segment. A myelin sheath forms from Schwann cells and surrounds the axon, except at its ending and at the nodes of Ranvier. Terminal buttons (boutons) are located at the terminal endings. (Reproduced with permission from Excitable Tissue: Nerve. In: Barrett KE, Barman SM, Boitano S, Brooks HL, eds. Ganong’s Review of Medical Physiology, 25e New York, NY: McGraw-Hill; 2016.)

An excellent demonstration of this principle is comparing A-delta fibers and A-beta fibers. The sensations of pain and touch are both examples of afferent signaling with a few differences that can be explained in terms of the sensory nerves that propagate them. We will not discuss the various receptor types found on sensory nerve dendrites (free nerve endings) in this chapter. A-beta fibers are specialized afferent nerves that transmit touch sensation. On average, they are 10 to 20 microns in diameter and are fairly myelinated. In contrast, A-delta fibers, which are one specific type of pain fiber, are smaller, with an average diameter of 6 to 12 microns, and are thinly myelinated. It is of no surprise that action potentials propagated through an A-beta fiber thus have a much higher conduction velocity than those through A-delta fibers.4
How a nerve cell functions plays an important conceptual role when performing nerve conduction studies. It is important to remember it’s the organization of a nerve cell within the body dictates its function.
Anatomic studies of the brain and spine are more available than dynamic functional studies; however, recent functional magnetic resonance imaging (MRI) studies of brain activity with respect to chronic pain, for example, have revealed a vast network of cortical communication and activation not previously visualized in the literature. In addition, these studies have demonstrated regions and networks of the brain that remain functionally active in certain conditions such as chronic pain, even at rest.5,6 Decades earlier, clinicians in the field of behavioral studies postulated these neural networks as neural circuitry that can be rewired through training.6 For simplicity’s sake, we will be focusing on the anatomic consideration of the CNS, and for the purposes of this chapter we will briefly touch upon basic structures in the brain before discussing key elements of the spinal cord.
The brain is the site of sensory integration and processing. Our brain, encased within a protective skull, consists of approximately 86 billion neurons,1 fluid-filled ventricles, and an intricate vascular supply. The brain is divided into two hemispheres that communicate with each other through specialized structures, most notably the corpus callosum and anterior commissure. Each aspect of the brain has a specific function. We will divide the brain into a discussion of the cortex and the brainstem.
The cerebral cortex, though considered the “hard drive” of the brain, interestingly encompasses a relatively thin layer of neurons only 2 to 5 mm in thickness,8 and can be further divided into four distinct paired lobes: the frontal lobe, the occipital lobe, the temporal lobe, and the parietal lobe. Each lobe has an array of distinct functions. The cortex is responsible for our thought process, sensory integration, the storage of memory, and the execution and precision of tasks. It is useful to know that the cortex works in concert with the lower brain structures and rarely ever functions solely by itself. For example, cortical activity is reliant on excitation from the thalamus, a subcortical brain structure8 (Fig. 3–6).
Figure 3–6
The cerebrum. (A) Lobes of the cerebral cortex, named according to the skull bones under which they are located. (B) Functional classification of regions of the cerebral cortex showing the location of major functional areas. Selected examples of Brodmann’s brain areas are indicated (e.g., areas 1, 2, and 3 are in the primary somatosensory cortex; area 4 is the primary motor cortex; area 6 is the premotor cortex; area 8 is the frontal eye field; area 17 is the primary visual cortex; areas 41 and 42 are the auditory cortex; areas 22 and 39 encompass Wernicke’s language area; and area 45 is part of Broca’s speech area). (Reproduced with permission from Neurophysiology. In: Kibble JD, Halsey CR, eds. Medical Physiology: The Big Picture, New York, NY: McGraw-Hill; 2014.)

The frontal lobe encompasses the anterior one-third of the cerebrum and is generally the processing unit for memory, task completion, planning, and other executive functions. The frontal lobe lies anterior to the central sulcus of the cortex and in addition to regions devoted to executive function and planning, it houses the motor cortex. The motor cortex lies in the posterior one-third of the frontal lobe and is divided further into the primary motor cortex, the premotor cortex, and the supplementary motor cortex.8 These structures work in cooperation with other subcortical brain structures to produce meaningful movement. For example, the supplementary and premotor cortex, in conjunction with the primary motor cortex and basal ganglia, aid in producing coordinated patterns of muscle movement. Topographically, the more finite and intricate the muscle movement, the larger the area dedicated to that movement in the cortex. This is best represented when looking at the motor homunculus (Fig. 3–7). One can see that the intricate motor movement for mastication garners a much larger area of the cortex than does knee flexion. Motor signaling originates in this region of the cortex and travels to the spinal cord with various inputs from the cerebellum, basal ganglia, thalamus, and other various brainstem nuclei. These fibers then travel contralaterally from their hemispheric origin via the corticospinal tract to their end-target muscle groups. Neural activation for movement is quite complex, however, and can undergo a high degree of neuroplasticity with activation of various other regions of the brain, such as the parietal lobe in the setting of amputation or stroke.9
Figure 3–7
Arterial supply of the primary motor and sensory cortex (coronal view). Notice the location of the homunculus with respect to the territories of the cerebral arteries. (Reproduced with permission from Simon RP, Aminoff MJ, Greenberg DA: Clinical Neurology. 4th ed. New York: Appleton & Lange, 1999.)

In addition to simple muscle movements, regions of the frontal lobe motor cortex are involved in complex movements that control our ability to express ourselves as humans. This is especially true when these regions are associated with neural activity from other aspects of the cortex. For example, within the premotor cortex located in the inferior frontal gyrus lies the Broca’s area that, when damaged, prevents a person from effectively speaking whole words or complete full sentences even if comprehension of words and sentences is not impaired. Thus, this is a breakdown between thought and language ability10 and is conveniently called expressive aphasia or Broca’s aphasia (Fig. 3–8).
Figure 3–8
Location of some of the areas in the categorical hemisphere that are concerned with language functions. Wernicke’s area is in the posterior end of the superior temporal gyrus and is concerned with comprehension of auditory and visual information. It projects via the arcuate fasciculus to Broca’s area in the frontal lobe. Broca’s area processes information received from Wernicke’s area into a detailed and coordinated pattern for vocalization and then projects the pattern via a speech articulation area in the insula to the motor cortex, which initiates the appropriate movements of the lips, tongue, and larynx to produce speech. (Reproduced with permission from Learning, Memory, Language, & Speech. In: Barrett KE, Barman SM, Boitano S, Brooks HL, eds. Ganong’s Review of Medical Physiology, 25e New York, NY: McGraw-Hill; 2016.)

The parietal lobe is located behind the central sulcus and occupies the mid-to-posterior aspect of the cortex. The parietal lobe deals with various forms of sensory signaling and language processing. The somatosensory cortex lies within the anterior parietal lobe, and it encompasses the area immediately posterior to the central sulcus. This region is otherwise known as the lateral postcentral gyrus. As with the motor cortex, the somatosensory cortex of each hemisphere is responsible for sensation on the contralateral side of the body.
When discussing the sensory cortex as a whole, it is important to understand that the secondary cortices for sensation (including vision, taste, hearing, and smell) are found in various other cortical structures and not the parietal lobe. There are sensory association areas with portions located in different cortical lobes that receive motor and sensory information from various regions and analyze or synthesize the information to drive comprehension.8 For example, the parietal-temporal-occipital association area resides within the parietal, temporal, and occipital lobes and synthesizes visual and auditory sensory information before being stored as memory within the prefrontal cortex in the frontal lobe.
The temporal lobe is located laterally in each hemisphere adjacent to the lateral sulcus. The primary purpose of the temporal lobe is to process sensory information, whether emotional, visual, or language based, and provide comprehension or meaning. In addition, the temporal lobe houses the primary auditory cortex, receiving audible sensation, which is then processed into meaningful information in the adjacent areas. Located in the posterior aspect of the superior temporal gyrus is a region called Wernicke’s area, which is highly responsible for comprehension of language that is both spoken and written. The area lies adjacent to the primary auditory cortex where audible stimuli are received. With reference to visual language comprehension, visual association areas feed this information to the Wernicke’s area in the temporal lobe.
The occipital lobe lies in the posterior aspect of the cortex and is primarily involved in visual processing. This includes perceiving visual environments and objects, but also receiving visual representation of the written word. The occipital lobe would then need to work in concert with the temporal lobe for comprehension of what is written, as described earlier. It is important to reiterate this constant coordination between the different lobes to comprehend the outside world and thus interact with it appropriately. For example, prosopagnosia is a condition characterized by the inability to recognize faces. For visual recognition, we not only need appropriate functioning of the visual cortex in the occipital lobe, but also appropriate processing of that visual information, which normally takes place in the temporal lobe. Thus, prosopagnosia relates to damage of not only the medial aspect of the occipital lobe, but also within the medioventral surface of the temporal lobe. On the other hand, unilateral or bilateral solitary damage to the occipital lobe can result in visual field deficits without any damage to the eyes or optic tracts.
Below the cortex we find divisions of the brainstem, most notably the medulla, pons, and midbrain. Near the brainstem lie the cerebellum, thalamus, hypothalamus, and basal ganglia, which will be discussed later. The brainstem contains motor and sensory nuclei for the face and head in the same way that the spinal cord contains anterior and posterior gray horns. The brainstem contains many sensory nuclei (hearing, taste, balance); however, it also serves a highly important purpose of subconscious activity, including control of blood pressure and respiration. The medulla is the direct rostral extension of the spinal cord and resembles the spinal cord in organization and function. The pons lies rostral to the medulla and contains a large number of neurons that relay information from the cerebral hemisphere to the cerebellum. The cerebellum lies dorsal to the pons and the medulla and receives somatosensory input from the spinal cord, motor information from the cerebral cortex, and input about balance from the vestibular organs of the inner ear.
The cerebellum and basal ganglia are important for motor control despite the majority of motor activity originating in the cortex. They play a major role in sequencing and planned coordination of motor function, whereas the cortex is the site for the origin of motor function (Fig. 3–9).
The cerebellum controls motor sequencing and, to some degree, the coordination between agonist and antagonist muscles to produce smoother overall movement. It also relays information to the cortex to aid in the planning of the next desired motor action. The cerebellar vermis, a band of neural tissue down the center of the cerebellum, is responsible for control of muscle movements of the axial body, neck, shoulders, and hip.8 On either side resides the lateral cerebellar hemisphere of which the intermediate zone is concerned with controlling muscle contractions in the distal portions of the upper and lower limbs, while the lateral zones deal with discrete timing of motor movements. The cerebellum receives various tracts from the motor, premotor, and somatosensory cortex in addition to sensory information from the peripheral nervous system.8 Injuries to the cerebellum lead to uncoordinated motor movement disorders like dysmetria, ataxia, intention tremor, and even dysarthria.
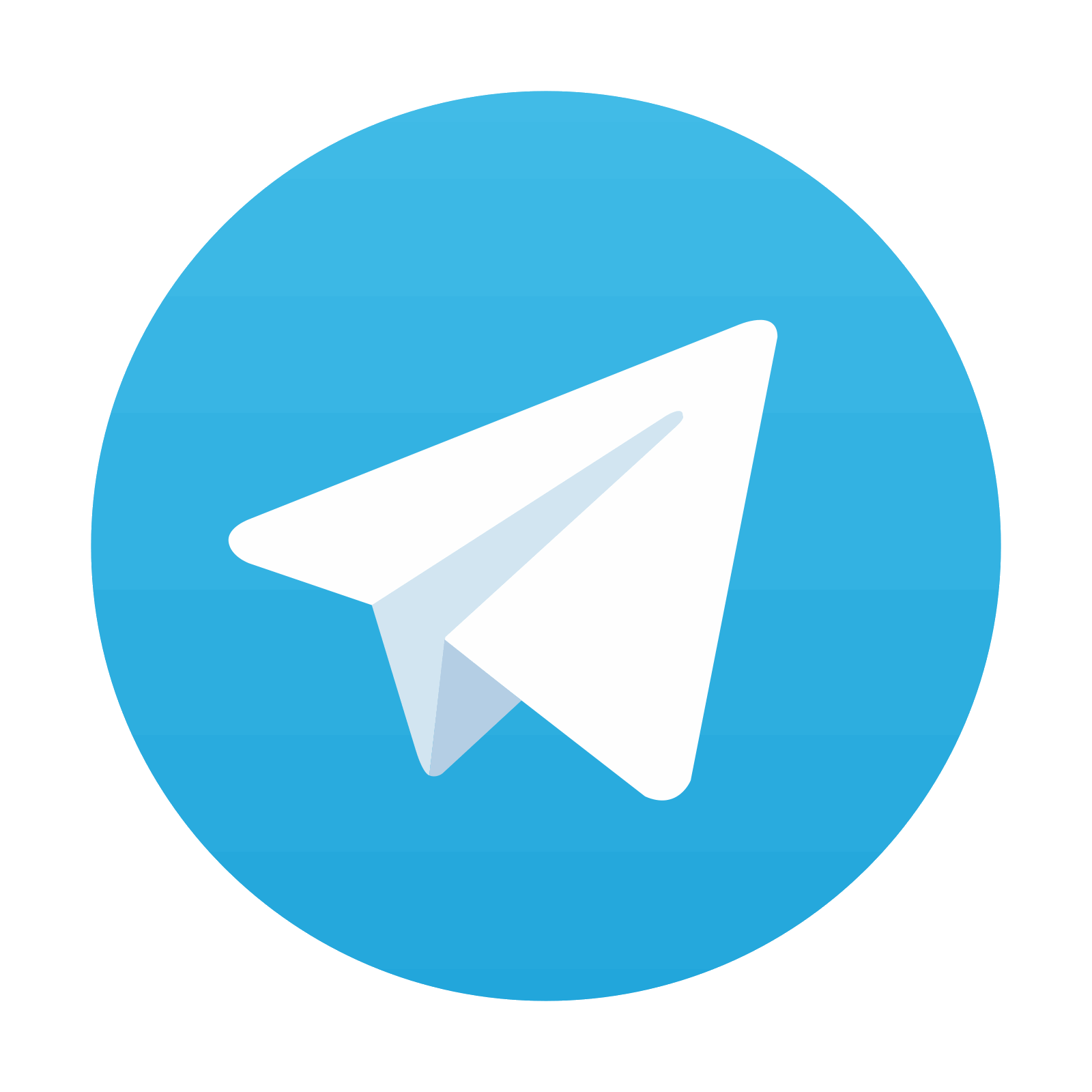
Stay updated, free articles. Join our Telegram channel
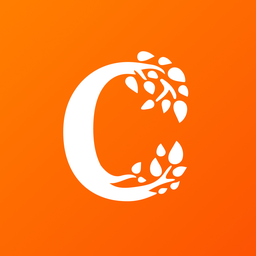
Full access? Get Clinical Tree
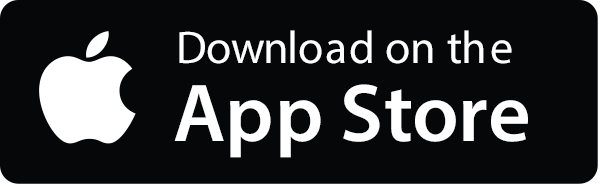
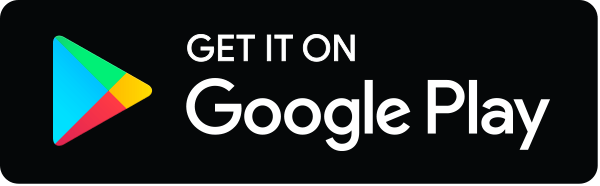