- ▪
Peripheral nerve end-organs require innervation to stay viable. The loss of the trophic stimulus of the nerve axon dooms the end-organ to atrophy and eventual death.
- ▪
Denervated muscles can be made to function by external electrical stimulation, and this can prevent some of the changes caused by denervation.
- ▪
Nerve injuries can result from many different mechanisms. They can result from compression by internal forces (e.g., tumors, fracture, callus) or by external forces (tourniquet or “crutch palsy”), or from ischemia, traction, x-radiation, inadvertent injection injury, or electrical injury.
- ▪
Regeneration of the peripheral nerve after injury is influenced by mechanical forces, delay to repair, the patient’s age, and the level of the injury.
- ▪
The nerve axon has the greatest likelihood of accurate regeneration when minimum scar is interposed between the severed nerve ends and if there is accurate alignment of the fascicles.
- ▪
The most commonly used technique for peripheral nerve microcoaptation is the epineurial repair technique.
- ▪
Electrophysiologic studies supported the use of grafts in lieu of coaptation under tension and showed excellent recovery even in the face of two suture lines.
There are many diverse causes of peripheral nerve dysfunction, and most treatments are outside the scope of the hand surgeon and therapist. Although some of the sequelae of nerve dysfunction can be treated surgically (e.g., tendon transfer or decompression), essentially only traumatic peripheral nerve injuries—transection, crush, compression, and stretch—can be repaired or reconstructed surgically. Because each particular type of nerve injury carries with it a different prognosis, it is important to fully appreciate the effects of each kind of nerve injury and the extent to which they affect the nerve cell, axon, and target organ. With this knowledge, the surgeon and therapist can offer the patient reasonable advice regarding prognosis and the expected results of surgical reconstruction. For a complete discussion of the basic science of nerve injury and repair, refer to Chapter 42 .
End-Organ Denervation Changes
As long as a nerve cell body remains alive, it has an unlimited potential for regeneration. This is not the case for the sensory or motor end-organ. Peripheral nerve end-organs require innervation to stay viable. The loss of the trophic stimulus of the nerve axon dooms the end-organ to atrophy and eventual death. However, this deterioration does differ in time for each kind of end-organ, and the understanding of the pathophysiology of end-organ degeneration will help determine the time constraints for surgical reconstruction.
Sensory End-Organs
The response of the sensory end-organ to denervation varies along the continuum from atrophy to frank degeneration and disappearance. This response not only is time dependent but also depends on the life cycle of the receptor in question. Muscle spindles, which undergo no further division after differentiation, respond to denervation by atrophy. Other cell types that are characterized by a short life cycle and high turnover—such as the taste bud—respond to denervation by degeneration and complete disappearance within 1 to 2 weeks. The integrity of sensory receptors apparently depends on an intact nerve fiber, although there need not necessarily be efferent impulses. Spinal ganglia, severed of their connections with the spinal cord, are able to maintain sensory structures as long as the axon is intact.
The three most common sensory end-organs—the Meissner corpuscle, the Merkel cell–neurite complex, and the Pacinian corpuscle—have similar responses to denervation. As a result of Wallerian degeneration, the axon terminal progressively degenerates over time and is absent by 9 months. The lamellar components of the Meissner corpuscles and the Pacinian corpuscles become atrophic but never completely disappear, as do the supporting structures of the Merkel cell–neurite complex. The Merkel cells seem to reduce in number, become atrophic, and possibly even become differentiated into transitional cells or keratinocytes after denervation. If the sensory receptor does proceed to complete degeneration after long-term denervation, it is lost to the receptor pool because the adult mammal appears to have lost the ability to form new sensory receptors de novo. , The muscle spindle, innervated with both motor and sensory fibers, responds in a similar fashion. When the γ-innervation is severed, the polar regions become atrophic, while the central sensory component (nuclear bag or chain regions) remains intact. When the dorsal roots, which supply the sensory portions, are injured, the central regions decrease in size, lose their equatorial collections of nuclei, and are eventually replaced by striated muscle.
Motor End-Organs
After the denervation of skeletal muscle, many changes are apparent. Clinically, the muscle ceases to function and there is gross muscle atrophy. Muscle atrophy after nerve injury results in a decrease of total muscle weight, a loss of total protein, and an overall reduction in muscle cross-sectional area. There is not a decrease in the number of individual muscle fibers, even though there is a relative increase in the amount of connective tissue stroma. Rates of atrophy can vary widely, with reports of as much as a 40% decrease in muscle mass within 1 to 3 weeks of denervation. This does not represent an irreversible degradation, however, and anecdotal reports have documented successful restoration of function after 22 years of denervation. Realistically, however, functional reinnervation is unlikely after 2 years of denervation. Denervated muscles can be made to function by external electrical stimulation, and this can prevent some of the changes caused by denervation. However, the role functional electrical stimulation plays in the reconstruction and rehabilitation of the peripheral nerve injury remains to be seen.
Mechanisms of Nerve Injury
Whenever a patient has sustained a peripheral nerve injury, the treating physician and therapist must fully understand the nature of the injury and the mechanism by which the nerve was injured. Each different type of injury carries with it a different prognosis, and at the time of acute injury, the clinical findings of numbness, paralysis, paresthesia, or pain shed little light on the pathophysiology and ultimate prognosis. By knowing the mechanism of the injury and the pathologic changes produced by that injury, the clinician may better predict the outcome and be better able to plan the care and ultimate reconstruction of the patient’s nerve injury.
Nerve injuries can result from many different mechanisms. They can result from compression by internal forces (e.g., tumors, fracture, callus) or by external forces (tourniquet or “crutch palsy”), or from ischemia, traction, x-radiation, inadvertent injection injury, or electrical injury. Although each mechanism represents a different type of injury, all of the injuries result from mechanical deformation, ischemia-induced metabolic failure, or both.
Mechanical deformation lesions encompass the entire spectrum of nerve injury, from first-degree neuropraxic injuries to fifth-degree injuries. In general, the effects of mechanical deformation depend on the rate of application of the deforming forces, the area of the nerve over which they are applied, and the magnitude of the forces. The regional anatomy of the nerve and its adjacent structures, as well as the nerve’s proximity to underlying bone and unyielding fascial bands, must be considered. Internal nerve anatomy also is important.
Peripheral nerves organized as a single fascicle are much more vulnerable to injury than nerves consisting of many fascicles surrounded by a larger amount of protective connective tissue. The mechanisms of injury creating mechanical deformation include acute and chronic compression, crush, and stretch. All of these lesions involve some degree of vascular injury. Occlusion of the supporting vasculature accompanies any deformation and may remain after the release of the deforming force. Recovery may depend on the degree of resultant ischemia that persists. When a peripheral nerve is subjected to a severe, abruptly applied deforming force, three grades of injury may result. There may be a rapidly reversible physiologic block, a local demyelinating block, or Wallerian degeneration. Although it is not completely understood which mechanism—mechanical deformation or ischemia—causes the injury, each factor has some role, and they are probably additive.
Acute Compression
Acute compression injuries are especially amenable to experimental examination and can be reproducibly created by the use of a pneumatic tourniquet. At low pressures (up to 30 mm Hg), impaired venular flow is observed and endoneurial fluid pressures are seen to be up to three times normal. Physiologically, decreased nerve conduction velocities are noted. At pressures of 60 mm Hg, nerve fiber viability is endangered by the creation of a local metabolic block, secondary to ischemia. In addition, at this level of compression, mechanical deformation within the nerve fiber is seen. At higher levels of compression (90 mm Hg and higher), there is even greater mechanical deformation of the axon and the supporting structures (Schwann cells), as well as collapse of the intraneural microcirculation, which is likely to persist upon the release of the deforming force. ,
Application of a tourniquet at pressures above systolic but at levels insufficient to cause cellular damage results in progressive centripetal sensory loss and paralysis within 30 to 40 minutes. In these first-degree neuropraxic injuries, the earliest pathophysiologic change is the inability of the nerve to transmit repeated impulses. Although the exact defect is unknown, ischemia from compression surely creates anoxic block of ionic and axonal transport. In addition, compression at high levels causes narrowing of the involved axons, increased endoneurial fluid pressure, and subsequent intraneural edema. , The classic examples of acute neuropraxic compression neuropathy include the transient conduction block, typified by paresthesias, that results from local pressure on a peripheral nerve. This causes the familiar sensation that one’s leg or arm “goes to sleep.” The conduction block rapidly recovers when the pressure is relieved or posture altered. When a motor nerve is involved, the sensation is one of “pseudocramps.” With extended tourniquet application at suprasystolic pressures, motor deficits and mild sensory losses occur. There is a higher degree of impairment in faster-conducting larger axons (primarily motor, proprioceptive, and light-touch fibers), whereas the smaller and nonmyelinated axons (pain, temperature, and autonomic function) are spared. Higher levels of compression yield a longer-lasting conduction block caused by focal demyelination without disruption of axonal continuity. This local conduction block is caused by mechanical deformation (nodal intussusception). , Higher levels of pressure on a nerve create further mechanical deformation, which results in the shearing of the mesaxonal Schwann cell from the adaxonal portion of the cell. This damages the myelin, which then degenerates and leaves an area of exposed axon. Conduction is not restored until remyelination is complete, and function returns in most cases by 3 to 6 weeks. Because axonal continuity is maintained, there is little, if any, target-organ degeneration. Because of the differential susceptibility of axons, complete paralysis (large fibers) can occur without loss of cutaneous sensibility (smaller myelinated and unmyelinated fibers).
Long periods of compression or high levels of pressure over a relatively small area of a peripheral nerve can produce a crushing injury that may be second, third, or even fourth degree. These are lesions-in-continuity, and the prognosis for recovery depends on the magnitude of the intraneural disruption. When the crush injury is axonotmetic, the axonal basement membrane remains intact, and regeneration progresses with an exact target-organ match and good recovery. Although recovery can be delayed, most patients have achieved 50% return by 4 months. When the crush injury is neurotmetic (third or fourth degree), function is mildly or moderately reduced by the failure of some axons to achieve a proper end-organ match. Axonal admixture as well as increased amounts of intraneural scar prevents complete return of function, and budding axons can become lost in the interposed scar and develop a neuroma-in-continuity.
Chronic Compression
Injury created by low-grade chronic compression differs from the acute compression injury in all aspects of etiology, histology, and clinical presentation. The susceptibility of peripheral nerves to chronic compression is a function of internal anatomy. Proximal nerves, which contain many fascicles and an abundant amount of supportive connective tissue, are much less vulnerable to compression than distal nerves. Within each nerve, peripheral fascicles are more affected than central fascicles, and within each fascicle, peripheral axons are more likely to be injured than the central ones. There is also a greater susceptibility to compression if the patient is afflicted with malnutrition, alcoholism, diabetes, or renal failure.
Histologic assessment of chronic nerve compression shows myelin sheath asymmetry, epineurial fibrosis, perineurial thickening, and, in severe stages, endoneurial fibrosis. Larger fibers appear to “drop out.” There is some Wallerian degeneration, and simultaneous regeneration of axons is seen. In contradistinction to the acute compressive neuropathy that creates nodal intussusception, the terminal loops of the inner lamellae of the thinned myelin near the entrapment become detached from the axon at the node and retract. An abundance of myelin appears at the opposite end of the internode, which produces bulbous paranodal swelling. The detachment and subsequent myelin retraction leave multiple consecutive internodes demyelinated. This partially accounts for the reduced conduction velocity seen in nerves injured by chronic compression. The conduction block seen in acute compression injuries is rarely seen in these chronic lesions.
Chronic compression also affects the vascular supply of the involved segment. In pressures as low as 30 mm Hg, impaired venular flow is observed, ultimately leading to congestion and anoxia. This induced ischemia leads to further vascular dilation, nerve swelling, and more compression, beginning a vicious cycle. The rapid reversibility of some chronic compression injuries supports the vascular etiology.
Axoplasmic flow is decreased in chronic compressive injuries, and even when the force is insufficient to create a demyelinating lesion, there is a profound effect on the function of the nerve. Distal to the compressive force, the nerve becomes more sensitive to low levels of pressure. Multiple subclinical levels of compression along any given nerve can lead to symptoms of compressive neuropathy. This double-crush syndrome implies that serial constraints of axoplasmic flow are additive in nature. ,
Histologic changes are observed in nerves subject to chronic compression. Epineurial fibrosis and perineurial thickening are noted, and there is a decrease in the number of large axons in the periphery of the affected fascicles. There is proteinaceous intraneural edema from the loss of the blood–nerve barrier, within which fibroblasts proliferate and ultimately render the nerve segment permanently scarred and potentially anoxic. Late chronic compression injuries are more likely to require extensive external neurolysis (and sometimes even internal neurolysis) than early lesions because of the extensive nature of the intraneural scar. Patients with chronic compression injuries often report the sensation of pain, but this can arise along any area of the affected nerve’s course and is often a misleading diagnostic sign. A more useful diagnostic sign that correlates with the site of compression is the Tinel’s sign. At surgical exploration, the affected nerve often is seen to be swollen, edematous, and hyperemic proximal to the area of compression. The nerve underlying the compression is often pale and narrow. At the time of surgical decompression, almost all patients obtain relief of their pain, although improvement in conduction delay takes weeks or months to return.
Stretch Injury
Peripheral nerves must incorporate within their structure the ability to accommodate changes in joint position. To do this, nerves have the inherent ability to stretch, recoil, and glide within their beds on their loose mesoneurial attachments. Under conditions of minimal tension, the nerve fibers assume an undulating course within the fascicle. As longitudinal tension is applied to the nerve, the nerve slides within the bed and begins to take up the load and become stressed. The epineurium—an elastic structure—begins to elongate by “stretch” and the fascicles within completely straighten out. Upon release of the longitudinal tension, the nerve recoils and resumes its natural resting length. As long as the nerve remains free to glide within its bed, significant stretch can be tolerated without injury. Normal excursions of nerves vary greatly, from a maximum of 15.3 mm (average for the brachial plexus) to a minimum of 1.15 mm (average for a digital nerve).
When a nerve is injured (through compression, scar, entrapment, or adhesions) and is subsequently anchored by scar tissue to its bed, it can be subjected to “overstretch” traction injury during normal physiologic demands. There are also certain traumatic states that can exceed the normal nerve excursion or limits of elasticity and therein create injury. A nerve achieves its strength through the perineurium. This layer has three orientations of collagen fibers in its outer sheath: circumferential, longitudinal, and oblique. , As longitudinal tension is applied the perineurium lengthens, but at the expense of the cross-sectional area. This creates an increase in the intrafascicular pressure along the entire length of the nerve. As long as the perineurium remains intact, the nerve maintains its elastic characteristics, but intraneural damage occurs far below the point of mechanical failure. The elastic limit, or allowable stretch limit, is about 20%. At that level there can be one or many areas of intraneural tearing, with axonal and fascicular disruption and areas of hemorrhage. Fibroblastic proliferation follows, which ultimately leads to intraneural scarring.
Although the true incidence of nerve stretch injuries is unknown, these injuries are commonly associated with traumatic events, such as fractures, dislocations, obstetric trauma, and occasionally inadvertent retraction during surgery. Of nerve injuries associated with fractures, 95% occur in the upper extremities, and of the five most commonly injured nerves, 58% are radial, 18% ulnar, 16% peroneal, 6% median, and 2% sciatic. In a prospective study of 648 nerve traction lesions in which the nerve was seen to be in continuity at the time of surgery, Omer reported that 70% achieved spontaneous recovery. In low-velocity gunshot wounds, 69% recovered after 3 to 8 months. Patients with high-velocity gunshot wounds also recovered from nerve lesions 69% of the time, but recovery required up to 9 months. In patients with nerve injuries associated with fractures and dislocations, 83% recovered spontaneously in 1 to 4 months, and in patients with nerve traction (stretch) injuries, 86% recovered in 3 to 6 months.
Not all traction injuries are traumatic. Some are the result of attempts to overcome an excessive nerve gap during reconstruction. This may be by stretch on the nerve stumps to achieve coaptation or by positioning the joints in flexion and creating the traction by the progressive postoperative extension of the joints. The progressive extension may lead to an ischemic injury or even exceed the elastic limits of the nerve. It has been shown that some length can be gained with slow stretch over time. This finding has been exploited using tissue expansion techniques that allow progressive nerve lengthening before nerve reconstruction to overcome nerve gaps. When caring for a patient with a major traction injury, we must be aware that the injury can affect the entire length of the nerve. The most clinically significant injury may be remote from the actual site of extremity injury, and this may test even the most astute diagnostician.
Ischemic Injury
The peripheral nerve requires a continuous and adequate supply of oxygen for aerobic metabolism to drive the normal functions of axoplasmic transport; maintain cell integrity; and remain primed for the generation, maintenance, and restoration of the membrane potentials necessary for conduction of impulses. To accomplish this goal, the nerve has an elaborate dynamic plexus of blood vessels composed of two integrated but functionally independent systems. The peripheral nerve can survive relatively long anoxic periods with a rapid recovery of function, but longer periods of acute ischemia or chronic hypoxia may produce irreversible injury. Muscle weakness, pain, paresthesias, hypersensitivity, and sensory deficits all are symptoms of ischemic nerve lesions.
Ischemic injury may result from three different pathologic processes. There may be large-vessel occlusion, arteriolar angiopathy, or nutrient capillary disease. Large-vessel occlusion caused by conditions such as trauma or embolism is amenable to medical management or direct surgical reconstruction. Arteriolar and capillary disease is indirectly approached by attempts at improving the nerve environment (e.g., flap reconstruction of the nerve bed) or by release of the offending perineurial and intraneural scar (or both). , Some of the pathologic states that affect the arteriolar (50 to 400 mm in diameter) vessels are necrotizing angiopathic disorders such as polyarteritis nodosa, rheumatoid arthritis, Churg-Strauss syndrome, Wegener’s granulomatosis, and thromboangiitis obliterans (Buerger’s disease). All of these disease states affect the epineurial arterioles and result in patchy occlusion and ischemic nerve damage.
The length of time the ischemic insult persists is the most important determinant of anoxic damage. Within the first 10 minutes of nerve ischemia, there is a rapid decrease in the membrane resting potential and electrical resistance. By 15 minutes, the action potential decreases and there is a further decrease in the resting potential, which blocks conduction. There is a complete loss in conductivity by 30 to 40 minutes. Reoxygenation brings recovery within 1 to 2 minutes, and recovery is usually complete by 10 minutes. This implies that the pathologic insult of ischemia is a metabolic phenomenon and not a morphologic one. In chronically ischemic nerves there is segmental demyelination, and irreversible axonal infarction may occur. , If regeneration occurs there is a favorable prognosis, because the intraneural destruction leaves the axonal basement membrane intact. This ensures directed axonal regrowth and proper nerve end-organ connectivity.
The question of tolerance to ischemia becomes very important when the issue of replantation or free-tissue transfer is raised. A normal nerve apparently can tolerate up to 8 hours of warm ischemia (room temperature) and suffer little morphologic damage. Nutrient blood flow is rapidly restored upon revascularization. After 8 hours there is a breakdown of the blood–nerve barrier, and the resultant influx of proteinaceous fluid negatively affects nerve regeneration by ultimately stimulating fibroblastic proliferation and subsequent intraneural scarring.
For more proximal amputations in which there is a significant amount of muscle involved, the tolerance to ischemia of the nerve is not of clinical importance, because the target organs suffer irreversible damage before the nerves. However, injured nerve fibers are more susceptible to induced ischemia than are normal nerves. This may be because there is a reduction in axoplasmic flow in the injured nerve (especially if severed).
Electrical Injury
An electrical injury to the upper extremity can run the gamut from minor to life-threatening and is associated with a significant percentage of resultant amputations (32.5%). The severity of these injuries depends on the current pathway and the relevant features of voltage level, tissue resistance, and current duration. The neurologic defects following electrical injury are usually immediate in onset and, for reasons incompletely understood, more commonly involve motor nerves. Most injured nerves show some recovery over time, but complete resolution of significant injuries is rare.
The major pathologic change in the electrically injured nerve is one of coagulation necrosis resulting from the generation of heat energy. The electrical current follows the path of least resistance, and resistance to flow increases in various tissues in the following order: nerve, blood vessel, muscle, skin, tendon, fat, and bone. , The electrical injury therefore preferentially follows the neurovascular bundles and creates deep-tissue destruction along these pathways. Flash thermal burns at the entrance and exit sites accompany these injuries. In 22% of reported cases of electrical burns, direct nerve destruction was the initial result of the injury. In nondestructive lesions, electrical injuries are found to cause an increase in threshold stimulus and a loss of amplitude of the response to supramaximal stimulation. Although some of these changes were reversible, the electrical injury left a persistent increase in latency and decreased conduction velocity. Severe injuries can lead to patchy necrosis of the entire nerve as well as central necrosis. Hemorrhage and subarachnoid bleeding also are common.
If the nerve is not destroyed, total demyelination in a multifocal distribution is seen, and blood vessels in the vasa nervorum sustain significant damage, thus creating a late ischemic injury. Complicating electrical injury are violent tetanic contractures that can result in hemorrhage, muscle rupture, and broken bones. Late changes principally result from chronic ischemic changes associated with vascular damage and progressive perineurial fibrosis. Unlike the previously mentioned vascular pathology, this chronic ischemic injury may respond to neurolysis and revascularization of the nerve (e.g., muscle flap, omental transfer). ,
Radiation Injury
As radiation treatment becomes more precise and refined, its use as a treatment modality in the therapy for cancer is increasing. With it come increasing survival rates for patients with cancer and also greater opportunity to observe the late effects of radiation injury on surrounding soft tissues and nerves. In the past, orthovoltage radiation (less than 1 million volts) was the standard, and this technique had very shallow tissue penetration. There were marked skin changes that limited the total dose of radiation before significant nerve damage could result. Today, megavoltage radiation (1 million to 35 million volts) has allowed increased penetrance to deeper tissue planes with minimal apparent skin damage, and the result is a much higher dose of radiation to the adjacent structures within the field.
Radiation injury to the peripheral nerve is poorly understood. Injury results from direct cell injury and indirectly from damage caused to the supportive vascular and connective tissues. These injuries are synergistic. Fortunately for neural tissue, there is relative stability in cellular population (i.e., there is little mitotic activity, and therefore little biologically significant damage occurs at the genetic encoding level) until attempts at neural regeneration. , Cellular damage is more pronounced if the radiation follows a nerve injury that stimulates the cellular supportive proliferation.
Radiation injury is permanent and does not seem to abate with time. Histologically, there is axonal dropout and patchy loss of myelin within the radiated segment. Attempts at Schwann cell proliferation yield a decreased total number of cells that produce abnormally thin myelin sheaths. An increased nerve cross-sectional area implies that there is persistent intraneural edema associated with abnormal endoneurial vessel permeability. Late examination reveals marked intraneural and perineurial fibrosis with apparent fibrous replacement of fascicles and a marked amount of thick pale scar.
Because of the common use of radiation in the treatment of cancers of the breast, brachial plexus radiation injury has been the most extensively studied type of radiation injury. The incidence of brachial plexopathy varies greatly, depending on the mode of delivery and the total dosage. In one series, using 4-MV radiation, 15% of patients developed neurologic symptoms after 5775 rads (5.775 Gy); after 6300 rads (6.3 Gy) the percentage increased to 73%. After radiation given by a 15-MV betatron, 22% of patients receiving between 400 and 5000 rads (0.4 and 5 Gy) developed an actinic plexopathy, increasing to 47% after 550 to 6600 rads (0.55 to 6.6 Gy). The latent period between radiation and the onset of symptoms of plexopathy has ranged from as short as 5 months to as long as 20 years, with a mean latency of 4.25 years. , Pain is by far the most common presentation of brachial plexopathy, with as many as 80% of patients reporting some amount. Fifty percent of patients describe their pain as severe. Sixty-six percent of patients presented with muscle weakness and atrophy, and this sign was often associated with marked upper extremity lymphedema. Most of the patients with sensory and motor deficits presented with median and ulnar involvement. ,
The typical patient who presents to the hand surgeon with upper extremity pain and who has had radiation for the treatment of a carcinoma presents a diagnostic dilemma. The problem is in distinguishing an actinic plexopathy from local recurrence or metastatic involvement of the nerves by tumor. Both present with like signs and symptoms and have the same mean onset. Both progress steadily over years, but progression of the plexopathy without the development of other metastatic sites is the best presumptive evidence for a radiation-induced lesion. In all surgical cases, absence of metastatic disease must be confirmed by liberal biopsy.
The strongest indication for surgical intervention in radiation-induced nerve injury is intractable pain, but surgery should not be approached in a cavalier fashion. Downgrading of function is a likely outcome, because the compromised nerves will not tolerate much surgical manipulation. The goals of surgical intervention should be to gently excise the strangulating fibrotic scar and to improve the vascularity of the involved nerves by transposition to an improved bed or by flap reconstruction.
Injection Injuries
The peripheral nerve is vulnerable to direct injection in many circumstances, and this can result in permanent damage to the nerve. It is common to inject various substances in the immediate vicinity of nerves when administering local anesthetic agents for regional anesthesia or injecting steroid preparations for the local treatment of inflammatory conditions. In addition, the intramuscular injection of materials such as antibiotics can result in inadvertent injection into an underlying peripheral nerve. Most of these injuries could be avoided by knowledge of the surface and underlying anatomy. When a nerve injection injury occurs, the patient can experience severe pain at the site of injection that radiates to the distribution of the nerve. This is often associated with a neurologic deficit—sensory, motor, or both.
Injection injury was thought to be caused by mechanical needle injury, allergic neuritis, ischemia, and the development of circumferential scar, as well as the intraneural deposition of neurotoxic substances. Several studies have shown that only the intraneural injection of neurotoxic substances causes significant nerve fiber injury. Only with diazepam, chlordiazepoxide, chlorpromazine, and benzylpenicillin was extrafascicular injection associated with nerve injury. The most severe injuries were related to the intrafascicular injection of meperidine, diazepam, chlorpromazine, hydrocortisone, triamcinolone hexacetonide, procaine, and tetracaine. Less severe, but still significant, injuries were produced by gentamicin, cephalothin, methylprednisolone, triamcinolone acetonide, lidocaine (worse if with epinephrine), and bupivacaine hydrochloride with epinephrine. Several of these drugs contain similar buffers, and these may be the offending agents. ,
Acutely, axonal dropout and Wallerian degeneration are seen. Alterations of the blood–nerve barrier change the normal endoneurial environment and may lead to late changes caused by the attendant swelling, ischemia, and intraneural scar. By 8 weeks after injury, there is severe intraneural fibrosis associated with minimal external scar. ,
When a peripheral nerve injection injury occurs, observation is indicated for the first 3 months, with electrophysiologic studies obtained about 6 weeks after the injury. Early surgical exploration with irrigation of the offending agent or external neurolysis is not recommended. Because the damage is intraneural, extraneural manipulations do not address the pathology. If there is no clinical recovery by 4 months, exploration is indicated. An internal neurolysis procedure should be done to decompress the scarred fascicles, and several months should be allowed to elapse to see what functional recovery follows. If little improvement is gained, one should perform resection of the neuroma incontinuity, followed by reconstruction.
Laceration Injury
Nerve laceration is likely to be one of the most common injuries that the peripheral nerve surgeon treats. Lacerations are either complete or partial, and all are fifth-degree neurotmetic lesions. A sharp instrument causes most such injuries, but some are associated with sharp bone fragments in a closed fracture. All are associated with a clearly defined neural motor or sensory deficit that will not improve without surgical intervention. Approximately 20% of all nerve lacerations that appear to be complete are in fact only partial lacerations, with contusion and stretch being responsible for the neuropraxic or axonotmetic deficit of the remaining intact fascicles. Nerve lacerations are essentially low-velocity crush injuries isolated to very small areas of the involved nerve. Even under ideal conditions, a divided nerve will have some component of crush injury in the proximal and distal stumps, which must be treated by careful debridement at the time of surgical reconstruction.
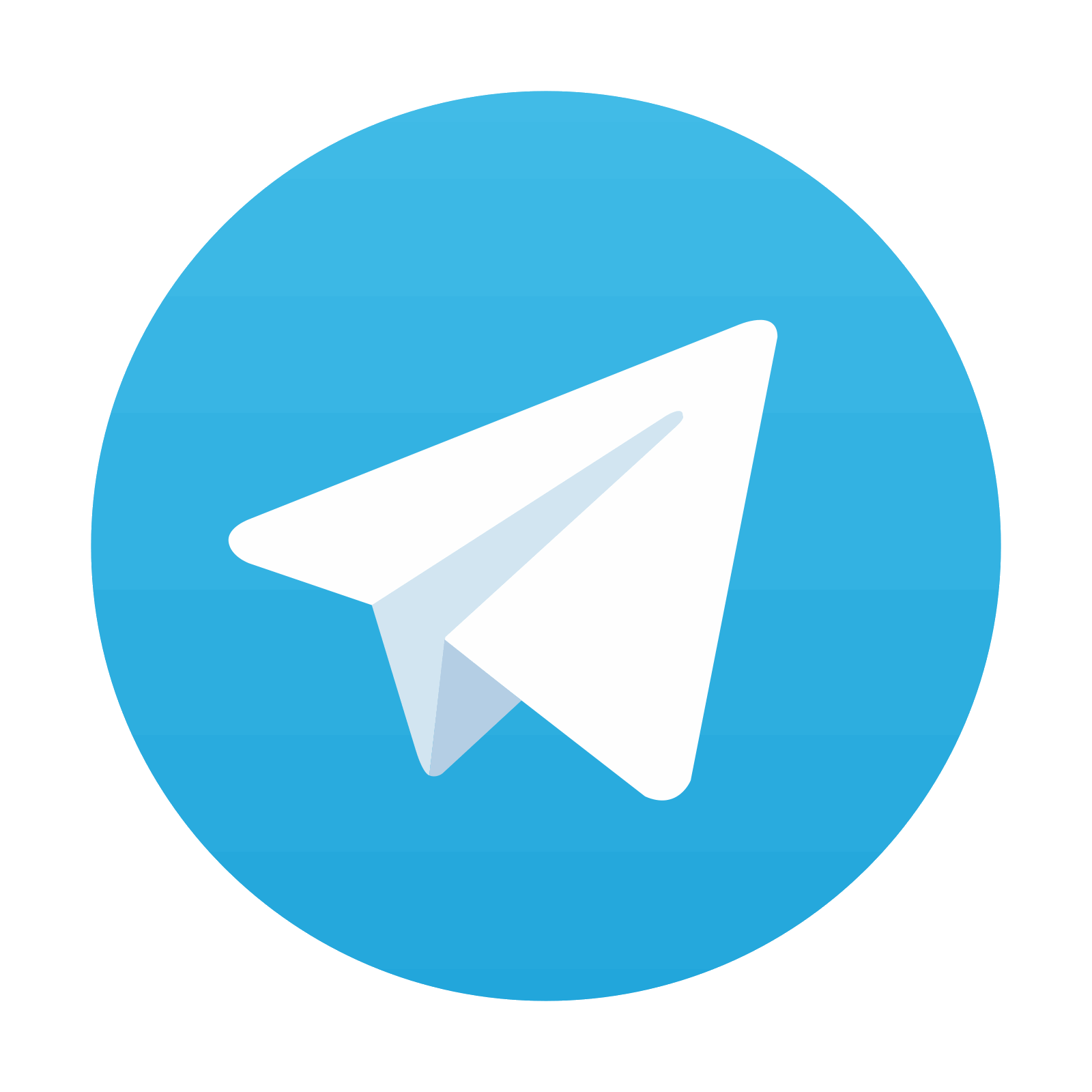
Stay updated, free articles. Join our Telegram channel
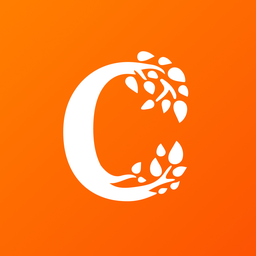
Full access? Get Clinical Tree
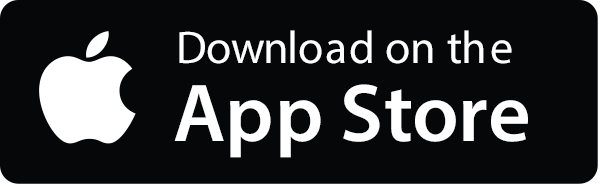
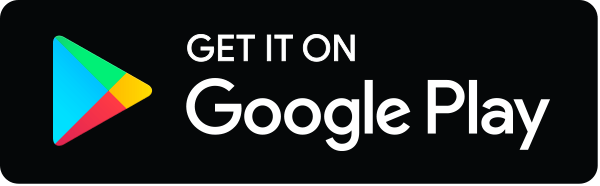