- ▪
Neuropathic pain is often a major component of neural tension dysfunction.
- ▪
The nervous system is designed to accommodate movement via excursion and strain, and excess strain can lead to compromise of the nervous system’s function.
- ▪
Neuropathology manifests as alterations in neurophysiology (symptoms) and mechanics (limitation of motion).
- ▪
The application of neurodynamic testing and treatment via neural mobilization requires a profound respect for the sensitivity of the nervous system to excess movement, especially in the diseased state.
- ▪
Treatment requires continuous and sound clinical reasoning when applying these techniques.
The use of neural provocation testing (NPT) and specifically the upper limb neural tension test (ULNTT) and nerve mobilization (NM) as an examination and treatment approach requires a clinician’s understanding of neural biomechanics and the consequences of neuropathology, pain, and movement dysfunction. Knowledge of treatment principles, guidelines, progression, precautions, and contraindications is essential for executing a safe and effective examination and treatment strategy. This is best summed up by the concept of neurodynamics developed by Michael Shacklock. It combines mechanical and physiologic properties of the peripheral nervous system that are dynamically interdependent and correlates the effects of tension and excursion on the peripheral nervous system. Attempting to separate these features clinically is nearly impossible. Alterations in neurodynamics will manifest as a pathomechanical (excursion, tension) and/or a pathophysiologic (pain sensory changes) problem. Shacklock now refers to this as neural tension dysfunction (NTD) that incorporates both of these concepts. Changes in neural physiology and mobility may result in the development of the patient’s symptoms and the limitation of motion confirmed via neurodynamic evaluation. This concept of neurodynamics also applies to treatment to restore normal neural physiology and mobility and alleviate pain originating in the nervous system.
Neuropathic Pain
Using NPT and NM mandates that the clinician understand that the vast majority of patients with NTD present with neuropathic pain as a primary feature. The International Association for the Study of Pain defined peripheral neuropathic pain as “pain initiated or caused by a primary lesion or dysfunction in the peripheral nervous system.” The mechanisms of neuropathic pain are not fully understood as evidenced by the list of reported mechanisms compiled by Allen in Box 118-1 . In the normal pain state, afferent (nociceptor) and central nervous system (spinal cord, thalamic, cortical) hyperexcitability occurs. Neuropathic pain may be a dysfunction of this process. For successful use of these examination and treatment techniques, the therapist must appreciate the interaction between the patient’s pain and accompanying limitations in motion.
Ectopic impulses
Neurogenic inflammation
Gene-regulated C-fos changes
Primary afferent nociceptor neuropeptide changes
Ephaptic connections
Sympathetic dysfunction
Neuronal sprouting: peripheral and central
Central sensitization
Thalamic low-threshold spike bursts
Nervi nervorum
Possible theoretical neuropathic pain mechanisms compiled by Allen.
It appears that the concept of abnormal impulse-generating sites (AIGSs) plays a key role in neuropathic pain. These sites are sources of ectopic stimulus generation or discharges related to an alteration in ion channel function and number. These ion channel changes usually occur at the site of demyelination, which can occur when a nerve is damaged by trauma or disease. This damage may result in the formation of microneuromas in continuity. The pain may arise from impulses in demyelinated, damaged, or regenerating afferent fibers. A number of different stimuli, such as temperature, cytokines, catecholamines, and metabolic and mechanical factors, can stimulate an AIGS. AIGSs will eventually cause changes in normal function of the dorsal root ganglion and neurons in the central nervous system (dorsal horn, thalamus, and cortical neurons), known as central sensitization. Pain from AIGSs has also been described as dysesthetic pain.
Neuropathic pain can also be attributed to nerve trunk pain, which may result from increased activity in nociceptive endings in the connective tissue of the peripheral nerves called nervi nervorum. Asbury and Fields theorize that nociceptive activity causing nerve trunk pain is created by chemically mediated increased mechanosensitivity of the neural tissue. This theory is also supported by Sauer and associates. These researchers reported that the nervi nervorum are responsible for the release of calcitonin gene–related peptide (CGRP), leading to an inflammatory cascade within the nerve. Because of this increased sensitivity, the application of an adequate stimulus, tension, and compression will result in a noxious response.
One final theory to consider is the direct effect of chemical sensitization of the peripheral nervous system. The chemical may be of non-neurogenic origin as a result of injury to connective tissue. Endogenous chemicals such as bradykinin, serotonin, histamine, prostaglandins, and leukotrienes are released and have been shown to affect nociceptive afferents. These endogenous chemicals may also be of neurogenic origin and are called neuropeptides and include substance P, CGRP, vasoactive intestinal peptide, and enkephalins. These chemicals are released by primary afferent neurons as a result of chemical or physical damage to the peripheral nociceptive afferent. These chemicals will result in the formation of neurogenic inflammation and potentially produce neuropathic pain. The result of this neurogenic inflammation is increased mechanosensitivity of the nervous system. As discussed later in the chapter, this increase in mechanosensitivity plays a role in the neural hyperalgesia experienced by patients in the form of antalgic posturing, mechanical tension, or compression via palpation.
Neuropathic pain presents with a variety of symptoms that are summarized in Box 118-2 . Patients with neuropathic pain often present with continuous and spontaneous pain that may not be related to any particular stimulus and may linger after the removal of an evoking stimulus (hyperpathia). The existence of spontaneous discharge has been confirmed in animal studies by Eliav and colleagues and Bove and colleagues in the presence of induced neurogenic inflammation. This neurogenic inflammation and increased mechanosensitivity may also be responsible for the distant projection of pain or its sometimes widespread nature. Neuropathic pain may be perceived as deep (cramping, aching, throbbing) or superficial (burning, pinching, stabbing). There often is a delayed response to mechanical stimuli, which may occur after repeated stimulation. Paroxysmal pain may also occur and is described as electric shock–like or shooting. Spontaneous ectopic discharge and lowered threshold mechanoreceptor function may be responsible for some of the bizarre symptoms reported by patients with neuropathic pain. Pain may radiate from a focal point along a continuous track or may be referred to other areas described as clusters or clumps of pain. The specific evaluation of neuropathic pain has been postulated by Galer and Jensen using the Neuropathic Pain Scale (NPS). The scale is designed to measure the distinct qualities of neuropathic pain. Preliminary testing shows the NPS to be discriminant and have predictive validity. Further testing of this scale is needed to confirm its usefulness in evaluating neuropathic pain. The reader is referred to Chapter 113 for a more detailed discussion of pain.
Allodynia: Nonpainful stimulus provokes pain
Hyperalgesia: Increased response to painful stimulus
Hyperpathia: Increased response to painful stimulus continues after it is withdrawn
Dysesthesia: Unpleasant abnormal sensation (spontaneous or evoked)
Biomechanical and Physiologic Concepts
Nervous System Continuum
The nervous system is a continuum. The peripheral, central, and autonomic nervous systems all combine to form one system that interacts as a unit of input and output. This continuum is achieved mechanically, electrically, and chemically. Figure 118-1 is an anatomic prosection demonstrating this concept visually prepared by Dr. Rufus Weaver and displayed in 1893 at the Columbian Exposition in Chicago. This anatomic preparation demonstrates how placing tension or strain on either the peripheral or central nervous system could have a potential effect on the nervous system in another location. For example, the ULNTT alters neuraxial or meningeal tension and provides the clinician with screening maneuvers to examine the irritability of the patient’s nervous system and its accompanying interfacing tissues.

Strain
The nervous system as a continuum requires mechanisms for elongation, tension, and glide. Sunderland and Bradley examined the mechanical properties of the peripheral nerve and nerve root to investigate strain failure rate. They reported that the elastic limit in the peripheral nerve varied 7% to 20% and failure strain varied 7% to 30%. For the nerve root, the maximal elastic limit was less than 15%, and failure strain occurred at 25%, indicating that the nerve root failed at lower loads than the peripheral nerve. Haftek investigated the effect of slow and quick stretch on albino rat tibial nerves. He reported that the initial process of elongation did not affect the nerve fiber but was physiologic in nature, described as unfolding. Progressive strain to failure demonstrated that histologic rupture of the epineurium occurred first. Before epineural rupture, damage in the form of neurapraxia or axonotmesis occurs. These strain levels are much higher than the clinician would want to impart when performing NPT or NM.
The strain and/or stress that occur along the course of the nerve as a result of upper extremity joint motion must also be considered. Strain is the change in length that occurs in a nerve as a result of unfolding in response to extremity movement. Millesi and colleagues, Zoech and colleagues, and most recently Wright and colleagues and Kleinrensink and colleagues demonstrated in cadavers that motion of the upper extremity results in stress being imparted along the entire course of the nervous system as measured by strain. Kleinrensink and colleagues, studying the effect of the ULNTT of strain on the peripheral nerves, determined that motion at the wrist increased the strain at the cord level of the brachial plexus. In addition, contralateral lateral flexion of the cervical spine increased strain in the cords of the brachial plexus and the three major nerves in the arm. Finally, the ULNTT of the median nerve was the most sensitive for the three major nerves and the most specific for the median nerve compared to the other ULNTT for the ulnar and radial nerves. Research performed in animal, cadaver, and limited human models verifies that the nervous system has multiple mechanisms to attenuate strain, tension, and elongation and that upper extremity and spinal motion can affect tension throughout the nervous system.
Tension within the nerve can also affect intraneural blood flow and nerve function. Lundborg and Rydevik determined that lower limits of strain (5%–10%) demonstrated the first signs of changes in blood flow in the epineural and perineural vessels. The upper stretch limit was 11% to 18%, causing complete occlusion that resolved after relaxation of the nerve. Using rabbit sciatic nerve, Ogata and Naito found that strain limits greater than 15.7% resulted in complete ablation of blood flow to the nerve. Complete ablation of blood flow also occurred when external compression was greater than 50 to 70 mm Hg. Studying the effect of strain on rat tibial nerve function, Kwan et al. reported strains of 6% or greater resulted in a 60% decrease in compound nerve action potential (CNAP) after 20 minutes. They concluded that longstanding low stress could affect the functional properties of the nerve.
In 1992, Wall and associates, using rabbit tibial nerves and measuring nerve conduction, also determined that strain rates of 6% or greater resulted in a 70% decrease in CNAP after 20 minutes. Recovery occurred to within 10% of prestretch values when the load was removed. At 12% strain, there was a rapid reduction in CNAP with complete conduction block after 50 minutes. There was only a 40% recovery once the load was removed. It was their opinion that mechanical deformation contributed to decreased nerve conduction and ischemia. Wall and colleagues concluded that the response to stretch might not be immediate; however, prolonged stretch may cause irreversible damage. Porter and Wharton examined the effect of nerve function by occluding the nerve’s blood supply and measuring the irritability of a muscle innervated by that nerve. They discovered that irritability in muscle increased within 2 to 11 minutes after the blood flow was occluded. The role of repeated versus continuous strain on nerve function was studied by Watanabe and colleagues. The authors applied a continuous traction at 1, 2, 5, and 0 N of force and a repetitive traction at 60 and 120 cycles per hour to the brachial plexus of a rat. They determined that there was no change in blood–nerve barrier permeability, functional grip strength, and electrophysiologic function, as measured by CNAP. In contrast, repetitive traction resulted in significant changes in all three measures with the higher repetitions (120 cycles per hour) causing the greatest change. The practical application of this information is that it supports that maximal strain rates using the ULNTT and NM should be less than 4% to 6%. Repetitive applications or oscillations of NM may result in damage to the nerve. The clinician must rely on the patients’ response (pain/paresthesia) to determine the amount of strain because it cannot be measured clinically.
The nervous system’s ability to accommodate tension is a product of an intraneural and extraneural anatomic design. Internally, the nerve is designed with undulations creating a tortuous nature. The nerves’ connective tissue layers form these undulations and were described by Clarke and Bearn when investigating the presence of the spiral bands of Fontana. These bands are present in the relaxed state of the nerve and disappear as tension is applied. A second mechanism that the nerve uses to tolerate elongation is intraneural gliding. The unique framework of the nerve’s connective tissue allows intraneural excursion between individual nerve fibers and their surrounding endoneurium and the endoneurium surrounding each nerve fiber. The epineurium allows excursion to occur between it and the perineurium of each fascicle. Finally, the nerve’s internal ability to tolerate tension and permit elongation results from an intrafunicular plexus formation described by Sunderland.
Excursion
Extraneural gliding provides for attenuation of tension via a gliding surface between the paraneurium and the epineurium. Extraneural excursion or gliding has been demonstrated in the central nervous system and the peripheral nervous system.
Central Nervous System
In the central nervous system, Reid demonstrated that 1.8 cm of excursion occurred in the spinal cord when performing movements of cervical and lumbar spine flexion and extension. He reported that 11.3% strain occurred in the cervical spine with the greatest amount of strain between the C2 and C5 levels. This increased to 17.6% when C2–T1 levels were combined. Movement of the nerve root that occurred was transmitted via the dural sheath and dentate ligaments and not directly to the rootlets. O’Connell demonstrated that cervical flexion caused excursion of the cord in the cephalad direction with increased nerve root tension. Cervical extension caused movement in the opposite direction and decreased nerve root tension. Cervical spine flexion caused 5 mm of excursion in the cervical region, 4 mm in the thoracic region, and 1 mm in the lumbar region. Straight leg raising and prone knee bend created caudal excursion of the spinal cord and increased nerve root tension at the lumbar level. Finally, Lew and colleagues, examining baboon cadavers, also reported that cervical flexion caused cephalad movement of the spinal cord.
Peripheral Nervous System
Neural excursion has also been demonstrated to occur in the peripheral nervous system. McLellan and Swash demonstrated that the median nerve underwent an excursion of 7.4 mm distally and 4.3 mm proximally with wrist and finger motion and elbow flexion/extension. In 1986, Wilgis and Murphy measured excursion of the brachial plexus, median, ulnar, and radial nerves in 15 cadaver arms. The greatest excursions occurred at the brachial plexus level (15.3 mm) with movement of the shoulder, the median nerve wrist level (proximal 14.5 mm and distal 6.8 mm), the ulnar nerve wrist level (13.8 mm), and 6.8 mm distal excursion of the ulnar nerve with elbow extension to flexion. Using fresh frozen cadaver specimens, Wright and colleagues examined excursion of the median, ulnar, and radial nerves. A summary of their findings is presented in Tables 118-1 through 118-3 .
Direction | Wrist | Elbow | ||||
---|---|---|---|---|---|---|
Average Distance | Joint | Motion (degrees) | Average Distance | Joint | Motion (degrees) | |
Distal | Shoulder | Abd 30 | Shoulder | Abd 30 | ||
Elbow | EF 90 | Elbow | EF 10 | |||
24 mm | Forearm | 15 mm | Forearm | Pron 60 | ||
Wrist | Ext 60 | Wrist | Ext 60 | |||
Finger | Ext 35 | Finger | Ext 35 | |||
Shoulder | Abd 110 | Shoulder | Abd 110 | |||
Elbow | EF 10 | Elbow | EF 90 | |||
Proximal | 12 mm | Forearm | 15 mm | Forearm | ||
Wrist | Flex 60 | Wrist | Flex 60 | |||
Finger | Flex 35 | Finger | Flex 35 |
Direction | Wrist | Elbow | ||||
---|---|---|---|---|---|---|
Average Distance | Joint | Motion (degrees) | Average Distance | Joint | Motion (degrees) | |
Distal | Shoulder | Abd 30 | Shoulder | Abd 30 | ||
Elbow | EF 10 | Elbow | EF 90 | |||
13 mm | Forearm | Pron | 11 mm | Forearm | Pron | |
Wrist | Ext 60 | Wrist | Ext 60 | |||
RD | RD | |||||
Finger | Ext 35 | Finger | Ext 35 | |||
Shoulder | Abd 110 | Shoulder | Abd 110 | |||
Elbow | EF 90 | Elbow | EF 10 | |||
Proximal | 11 mm | Forearm | Sup | 5 mm | Forearm | Sup |
Wrist | Flex 60 | Wrist | Flex 60 | |||
UD | UD | |||||
Finger | Flex 35 | Finger | Flex 35 |
Direction | Wrist | Elbow | ||||
---|---|---|---|---|---|---|
Average Distance | Joint | Motion (degrees) | Average Distance | Joint | Motion (degrees) | |
Distal | Shoulder | Abd 30 | Shoulder | Abd 30 | ||
Elbow | Flex 90 | Elbow | EF 10 | |||
7.6 mm | Forearm | Sup | 2.8 mm | Forearm | Pron/Sup | |
Wrist | Flex 65 | Wrist | Flex 65 | |||
UD 30 | UD 30 | |||||
Finger | Ext 0 | Finger | Flex | |||
Shoulder | Abd 110 | Shoulder | Abd 110 | |||
Elbow | Ext 10 | Elbow | EF 90 | |||
Proximal | 1.8 mm | Forearm | Pron | 11.4 mm | Forearm | Sup 30 |
Wrist | Ext 60 | Wrist | EF 0 | |||
— | R/UD 0 | |||||
Finger | Ext 0 | Finger | EF 0 |
Wright and associates demonstrated that the average median nerve distal excursion measured at the wrist was 24 mm and occurred when the upper extremity was positioned in shoulder abduction of 30 degrees, elbow flexion of 90 degrees, and wrist/finger extension. Average proximal median nerve excursion measured at the wrist was 12 mm with the shoulder abducted 110 degrees, elbow extension of 10 degrees, and wrist/finger flexion. Excursion of the median nerve measured at the elbow revealed that an average distance of 15 mm occurred distally with shoulder abduction of 30 degrees, elbow extension of 10 degrees, and wrist/finger extension. Proximal excursion averaged 15 mm with 110 degrees of shoulder abduction, elbow flexion of 90 degrees, and combined wrist and finger flexion.
The average excursion of the ulnar nerve measured at the wrist was 13 mm distally with shoulder abduction of 30 degrees, elbow extension, pronation, and wrist/finger extension. The average proximal excursion was 11 mm with shoulder abduction of 110 degrees, elbow flexion of 90 degrees, supination, and wrist/finger flexion. Measured at the elbow, the average distal excursion was 11 mm with shoulder abduction of 30 degrees, elbow flexion of 90 degrees, pronation, and wrist/finger extension. Proximal excursion of 5 mm occurred with shoulder abduction of 110 degrees, elbow extension of 10 degrees, supination, and wrist/finger flexion. The total distance necessary to accommodate upper extremity motion was 24 mm at the wrist and 17 mm at the elbow. The total motion required to accommodate upper extremity motion was 36 mm at the wrist and 30 mm at the elbow.
The radial nerve demonstrated less excursion than the median or ulnar nerve. A total of 11.4 mm of proximal excursion occurred at the elbow in the position of shoulder abduction of 110 degrees, elbow flexion of 90 degrees, and distal joints at 0 degrees. Distal excursion measured at the elbow was 2.8 mm with shoulder abduction of 30 degrees, elbow extension, and wrist/finger flexion. Excursion measured at the wrist was 1.8 mm in the proximal direction with the shoulder in abduction of 110 degrees, elbow extension, and wrist/finger extension. The greatest distal excursion (7.6 mm) occurred with the shoulder in 30 degrees of abduction, elbow flexion of 90 degrees, with the wrist flexed and fingers extended.
In vivo studies using ultrasonography confirmed the presence of nerve excursion. Dilley and colleagues reported total median nerve excursion of 10.4 mm in the upper arm and 4.2 mm in the forearm with elbow extension as the moving component. They also confirmed the concept of the nervous system continuum by demonstrating that the distal motion affects the nerve proximally. Passive wrist and index finger extension also result in similar median nerve excursions of up to 4.5 mm. Finally, the excursions that occur in vivo are less than those that occur in the cadaver studies previously mentioned. From these data, it can be seen that changing the position of the shoulder, elbow, wrist, and fingers can affect nerve excursion distally or proximally. These central and peripheral nervous system studies support the continuum theory, which explains the nerve tensioning and gliding that is imparted with the ULNTT and NM.
An integral component in understanding peripheral nerve excursion is appreciating that the interfacing tissues surrounding the nerve along its entire course are also required to adapt in length in relationship to joint motion. Millesi and colleagues reported that the median nerve bed must adapt by as much as 20% in length at the elbow and wrist to accommodate motion. Zoech and colleagues specifically investigated the difference in the length of the median nerve bed in positions of maximal flexion and extension of the upper extremity. They demonstrated that maximal extension required a 4.3% change in length, and flexion resulted in as much as a 14.9% decrease in overall length. Therefore, not only must the peripheral nerve be able to adapt to elongation and tension, but the interfacing tissues that form the nerve bed must also adapt independently to changes in length resulting from joint motion. This same adaptation of the nerve bed occurs within the central nervous system.
Neural Vasculature and Movement
In addition to the peripheral nervous system’s ability to accommodate movement, its physiology and function are also dependent on its vascularity and the maintenance of a pressure gradient system. This system allows for the maintenance of vascular perfusion and physiology and is best depicted by Sunderland’s model as shown by the formula PA > PC > PF > PV > PT. This model requires arterial pressure (PA) to be greater than capillary pressure (PC), which is greater than fascicular pressure (PF), which exceeds venous return pressure (PV) and ultimately is greater than tunnel pressure (PT). Alterations of any one of these five pressures have the potential to affect circulation and axoplasmic flow throughout the nerve. Alterations in tunnel pressure often result in the more common peripheral nerve entrapments in the upper extremity such as cubital tunnel syndrome, ulnar tunnel syndrome, and carpal tunnel syndrome (CTS).
Pechan and Julis, examining ulnar nerve pressures at the elbow, demonstrated a twofold increase in cubital tunnel pressure with cervical spine and shoulder motion and a sixfold increase with ulnar nerve provocative testing (elbow flexion, wrist extension, and the arm above the head). This study further supports the concept that remote motions can have an influence on not only neural motion and strain but also neural pressures and vascular flow. Studying the rabbit vagus nerve, Dahlin and McLean verified a conduction block with 30 mm and 50 mm of external compression sustained for 2 hours was reversible within 24 hours. At 200 mm of external compression for 2 hours, reversal took as long as 3 days, and at 400 mm, reversal took 7 days. Nemoto and colleagues clamped a dog sciatic nerve at one of two locations proximal and distal to the experimental compression site. Their work established that two low-grade compressions along the nerve trunk created greater damage than a single compression and the damage from dual compression exceeded the expected damage caused by an isolated compression. This supports the theory of double crush described by Upton and McComas. In addition to affecting vascular flow, axoplasmic flow is also altered with less external pressure than occurs with minor CTS.
Taken collectively, these general principles emphasize the key point—that the nervous system is designed for movement. As with any other soft tissue structure, when the nervous system is in a diseased and hyperirritable state, mechanical stresses such as compression and tension may provoke pain syndromes and movement dysfunction associated with the nervous system and its interfacing tissues. The clinician should maintain awareness that not only is direct and local tissue affected, but other tissues innervated by the involved nerves may also be the source of the patient’s pain and movement dysfunction.
Neuropathology and Its Manifestations
Neuropathology
Although it is not the intent of this chapter to discuss neural pathology in detail, the neuropathologic consequences on the mechanical properties, vascular flow, and axoplasmic flow of the nerve must be considered when using the ULNTT and NM (gliding, sliding, or tensioning) techniques for treatment. These three factors contribute to the cause of hyperirritability of the peripheral nervous system and its interfacing tissues. The vascular system may be compromised by external compression or adverse tension. NTD may be the result of adaptive shortening of the peripheral nerve from positioning or scarring of the nerve (intra- or extraneural). Compromise of the nerve’s vascularity can lead to a state of inflammation in or around the connective tissues of the nerve, increasing its level of irritability. Vascular changes may also lead to alterations in neurovascular dynamics and intraneural fibrosis. External compression can lead to compromise in axoplasmic flow. This compromise reduces the transport of neural filaments, microtubules, and neurotransmitters along the axon to its terminal ending and the return of metabolic by-products, potentially altering the nerve’s physiology and function. As a result of this chemically mediated inflammatory process and/or the loss of intra- or extraneural gliding capabilities, mechanical irritability of the nerve will occur, resulting in repetitive forces being placed across the fixed (adherent) nerve segment. This loss in neural motion tolerance in one segment requires force attenuation to be achieved over a shorter segment of the nerve, exposing it to further damage or injury.
This neuropathologic process increases the nerve’s vulnerability throughout the upper extremity such as in the confined space of the cubital or carpal tunnel. The interfacing tissues that surround the nerve along its course also create these spaces or tunnels. Two examples of these are the median nerve passing through the pronator muscle or the radial nerve passing through the supinator muscle. Nerves are also more vulnerable at relatively fixed points where motion introduced at these levels minimizes the nerve’s capability of tolerating elongation forces. This frequently occurs where the nerve branches or is relatively fixed such as passing through fascia. Finally, the nerve is vulnerable to external compression whenever it rests across a hard surface such as the radial sensory nerve as it passes across the radius.
The final consequence of peripheral neuropathology is fibrosis. Occurring in two ways, intra- and extraneural fibrosis removes the nerve’s inherent ability to elongate or potentiate tension within the nerve fascicles and the gliding that occurs between the connective tissue layers of the nerve and its interfacing tissues. Intraneural fibrosis causes the loss of the tortuous course of the nerve or its undulations, resulting in the loss of internal glide and the unfolding capability of the nerve. Extraneural fibrosis limits the nerve’s ability to move within its nerve bed or between the interfacing tissues, creating mechanical interference. In either scenario, the lack of nerve mobility results in increased stress or strain delivered to a shorter nerve segment as joint motion occurs. This fibrosis may ultimately lead to the onset of pain and adaptive shortening of the nervous system, altering joint movement and extremity function.
Clinical Manifestations
Elvey, Butler, and Gifford and Butler have all described the clinical manifestations of this pathologic process. Simplified, these manifestations are pathophysiologic: symptoms reported by the patient such as paresthesia and pain and pathomechanical alterations in neural mechanics, which are limiting isolated or multisegmented motion of the upper extremity. Elvey also described the clinical response observed through a series of clinical examination techniques. These techniques result in provocation of the patient’s symptoms (pathophysiologic) and identifying motion dysfunction or limitations (pathomechanical) of the involved extremity, spinal segment, or both. Elvey and Butler describe a resistance encountered with passive extremity motion resulting from altered neural mechanics. Butler refers to these as “tissue barriers” encountered while performing the ULNTT. Elvey and Balster and Jull theorize that the resistance encountered results from hyperirritability of nervous tissue, causing a protective reflexive muscle contraction.
ULNTT and NM Research
Anatomic Relationships to the ULNTT
It is often assumed that the ULNTT and NM are neural tissue specific. These techniques are multisegmental and often involve mechanical deformation of other structures such as arteries, fasciae, and nerve roots and accompanying meningeal tissues. To confirm that there is a relationship with neural tissue, Edgar and colleagues studied the relationship of upper trapezius length and extensibility of neural tissues in normals. Using the ULNTT, they concluded that there was a relationship between upper trapezius and neural tissue extensibility. Subjects with less neural extensibility also have less upper trapezius length. In a cadaver study by Wilson and colleagues, strain in the lateral cord of the brachial plexus and segments of the subclavian artery was measured. They determined that applying the ULNTT resulted in strain in the lateral cord and the subclavian artery. Because of common sympathetic innervations, they theorized, the subclavian artery could be a potential source of symptom provocation leading to a false-positive result. Moses and Carman examined three cadaver specimens to determine whether the ULNTT was specific to neuromeningeal tissue. They concluded that the nerve roots of the lower cervical spine attach to innervated structures and that these tissues may also be stressed with the ULNTT, possibly causing a false-positive result.
Excursion
Additional cadaver studies have been conducted to examine neural excursion and its relationship to component movements of the ULNTT. Bay and colleagues demonstrated that wrist position (extension) plays a significant role in excursion with movement of the fingers (extension–flexion). Coppieters and Alshami confirmed that greater excursion occurred with a sliding technique, a two-component motion or moving two joints simultaneously, than with a one-component movement (one joint), and the least amount of excursion occurred with full tension technique (ULNTT).
Strain
There is anatomic evidence supporting that the ULNTT and NM also produce changes in strain in the brachial plexus and peripheral nerves. Lewis and colleagues studied five fresh cadavers to measure strain in the median nerve distal to the axilla with the application of the ULNTT. They reported a significant increase in tension with elbow and wrist extension, contralateral cervical lateral flexion, and ipsilateral straight leg raise. The importance of these findings is that remote motions increase tension in the nerves at locations distant to the motion. This is further evidence of the continuum theory of the nervous system.
Kleinrensink and colleagues reported additional evidence of strain within the brachial plexus and peripheral nerves. In three cadavers they investigated the strain of the brachial plexus and the three major nerves of the upper extremity while applying the ULNTT and a modification with cervical contralateral lateral flexion. Significant increases in strain in the nerves and brachial plexus occurred in all three tests. A specific ULNTT for each nerve also caused strain in the brachial plexus and the other peripheral nerves. These results question the specificity of each ULNTT and confirm the sensitivity. They concluded that the median nerve ULNTT was the most sensitive. These findings support earlier work that demonstrated that strain in the median nerve measured in the axilla and the proximal and distal forearm was increased even with remote joint positioning. These two studies support the work of Lewis and colleagues that remote joint motion will cause strain along the entire course of the nerve. Therefore, the clinical ability of the ULNTT or NM to isolate a particular segment of the nerve is difficult.
Additional cadaver studies confirmed increases in strain within the median and ulnar nerve. Byl and colleagues determined that maximum nerve strain occurred with the full median ULNTT: 8.2% ulnar nerve and 6.7% median nerve. Coppieters and Alshami also demonstrated increases in median nerve strain using six different components of the median nerve ULNTT. Maximum tension occurred with the full median nerve ULNTT measured at the wrist (4.7%) and above the elbow (4.2%). These results contradict one another in terms of the “safe zone” of strain in the nerve (4%–6%) to avoid compromise of blood flow and conduction. Finally, Coppieters and Butler determined that strain on the median nerve was significantly less when performing sliding movements compared with tension movements. All three tensioning techniques resulted in strains greater than 4%.
Normal Responses to the ULNTT
A number of authors have investigated the response of the ULNTT in normal subjects. Kenneally and colleagues reported on the response of the ULNTT applied to 100 normal subjects. In 80% to 90% of the subjects, the most common response was pain/stretch and/or paresthesia over the radial aspect of the forearm, thumb, and radial three fingers, occasionally the ulnar side of the hand, and a deep stretch sensation in the cubital fossa and palm. In 1991, Yaxley and Jull investigated the responses of 50 normal subjects to a modification of the ULNTT for the radial nerve. The most frequent response was a strong painful stretch over the radial aspect of the proximal forearm and upper arm, followed by stretch over the biceps. An increase in arm symptoms occurred in 90% of the extremities when cervical contralateral lateral flexion was added. The modified test of wrist/finger extension revealed a different response of pain over the medial elbow, anterior forearm, palm, and the middle three fingers or the entire hand excluding the thumb.
Other authors have investigated the neural and kinetic response to the ULNTT. Balster and Jull studied 20 asymptomatic subjects using surface electromyography, elbow goniometry, and pain perception in two groups of more or less extensible neural tissues. In the less extensible group, there was greater electromyographic activity and less elbow extension. Pain perception was not significantly different between the two groups. The result of this study is evidence of a reflexive muscle contraction tissue barrier in response to the application of the ULNTT and a possible correlation between the patient’s perceived pain and limitation of motion measured at the elbow. Coppieters and colleagues attempted to quantify this increased upper trapezius tone and limitation of motion by measuring the scapula elevation force and combined wrist/elbow motion using electrogoniometry in a pilot experiment with five asymptomatic (normal) subjects. They determined that there was a greater scapula elevation force in the ULNTT than in the nontension position. In a further study of 35 normal subjects, the ULNTT was applied in five test variations. As each variant was applied, there was an increase in the patient’s subjective response and a decrease in elbow range of motion. Coppieters and colleagues concluded that although neurodynamic testing assessed non-neural and neural structures, the high level of paresthesia supports that some of the response is neurogenic in nature.
In all these studies, the authors reported a high level of intrarater reliability for the application of the ULNTT. Because all these studies on normal subjects reported subjective responses, it makes confirmation of neurogenic involvement in the patient population more difficult and complex. Several of these studies also attempted to quantify the ULNTT. These results provide early evidence of the potential for objective measurement of neurodynamic testing and confirm the hypothesis of altered extremity mobility as a component of a positive neurodynamic test.
The use of high-frequency ultrasound images has demonstrated the effectiveness in assessing median nerve excursion in vivo. Dilley and associates examined ulnar nerve excursion during four different upper limb movements similar to components of the ulnar nerve ULNTT. They demonstrated that ulnar nerve excursion occurred in the forearm and to a lesser extent in the upper arm. Additionally, the amount of excursion was dependent on the wrist and shoulder position. Echigo and colleagues also used ultrasonography to examine median nerve excursion during nerve gliding exercises. Their study examined the component motions of passive wrist/finger extension and active finger flexion measuring median nerve excursion in the forearm. Passive wrist and digital extension resulted in distal nerve gliding of 1.9 mm in elbow extension and pronation and 3.0 mm in elbow flexion and supination. Proximal excursion of 0.8 mm occurred for active hook fist and 1.3 mm for active grasp. Coppieters and colleagues confirmed the earlier cadaver findings of Coppieters and Alshami. Using ultrasonography, they confirmed that more excursion occurred using the sliding technique than the tension technique and the difference was significant.
Specificity and Sensitivity
Cadaver studies have examined the sensitivity and specificity of the ULNTT. Recently, the specificity of neurodynamic testing has been examined using various sensory perception and experimentally induced pain models using a thermal pain sensitivity model in a quasi-experimental design in healthy subjects. Beneciuk and colleagues determined that the group treated with NM had a decrease in temporal summation, improved range of motion, and a decrease in sensory descriptors rating. The ULNTT alters the sensory perception threshold, and there is a weak but significant effect of age, with perception leaning toward hypoesthesia. In two separate studies using an experimentally induced pain model, the straight leg raise and slump test and the median nerve ULNTT did not affect the experimentally induced pain, indicating that the neurodynamic test is specific to the nervous system. Further support for the specificity of neurodynamic testing was provided by Boyd and colleagues when they confirmed the mechanosensitivity of the lower extremity nervous system using the straight leg neurodynamic test. Collectively, these experiments support the specificity of neurodynamic testing.
Clinical Research
Clinical research has been limited to reliability, validity, normal responses to the ULNTT, and responses of select pathologic groups. Viikari-Juntura investigated the interexaminer reliability of various cervical examination techniques including the ULNTT. A group of 52 patients were examined with four special tests including the ULNTT by two examiners before undergoing cervical myelography. The authors reported poor interrater reliability; however, the test was only performed on patients 17 through 52 and not as typically described. Additionally, only the patients’ response was measured with no consideration for any limitation of motion. Sandmark and Nisell investigated the validity of five common manual neck pain–provoking tests including the ULNTT. They determined that the ULNTT had a specificity of 94%, a sensitivity of 77%, a positive predictive value of 85%, and negative predictive value of 91% in 22 of 75 subjects randomly selected with reported neck pain. In a single case study, Shacklock examined the validity of the ULNTT in a surgically proven neuropathy of the ulnar nerve at the elbow. He reported a positive ULNTT result based on loss of motion in shoulder abduction of 10% compared with the contralateral side and an abnormal response based on Kenneally’s work.
Neurodynamic Testing Validity and Variables
Multiple authors have also investigated the presence of positive ULNTT results in patient populations. In a study of 60 patients with repetitive strain injury, Elvey and associates found that the ULNTT was positive in 59 of the patients for reproduction of their symptoms. In a study of 40 patients reporting pain and paresthesia associated with a neck injury, the ULNTT was performed and compared with 20 normal subjects. A positive test result was based on symptom provocation. Yeung and colleagues reported a sensitivity value of 0.9 and a specificity value of 1.0; however, the exact statistical method was not elucidated. Quintner, in a retrospective study, reported on 22 patients, all with positive findings on the ULNTT with a stretch-induced cervical–arm pain syndrome. Byng examined three different groups—patients with upper limb overuse syndrome (ULOS), asymptomatic keyboard users, and asymptomatic nonkeyboard users—and found that the ULNTT used produced positive results in 100% of the patients with ULOS and the asymptomatic keyboard users. Perhaps this indicates that the ULNTT may be useful in identifying those people that may have subclinical pathology. The ULNTT was also found to be useful in identifying and having diagnostic accuracy for those patients with cervical radiculopathy. Patient symptom provocation and reduced tissue extensibility (limitation of motion) were the criteria for a positive test result.
Assessment of variables other than symptom response to the ULNTT has also been measured. Yaxley and Jull, in a series of 20 patients with tennis elbow, used the radial nerve ULNTT with positive criteria of symptom response and the limitation of motion at the glenohumeral joint as determined by tissue resistance. They determined that glenohumeral motion (abduction) was significantly different on the symptomatic side based on wrist position. Selvaratnam and colleagues assessed range of motion for the ULNTT. In one of the few control group studies, they compared three groups of subjects: a group with a known brachial plexopathy, a group with sports injury, and an asymptomatic group of 25 subjects. A goniometric apparatus was used to measure upper extremity joint position and cervical motion while performing the ULNTT. The upper extremity joints were sequentially moved until the motion elicited pain or end range was achieved based on pain onset and tolerance. The authors found a significant difference in range of motion to sensory change between the brachial plexus group and the sports group with less motion and earlier onset of pain in the range of motion in the brachial plexus group. Similarly, there was a significant group interaction with a greater reduction of motion in the brachial plexus group than the asymptomatic group.
Other authors have also examined range-of-motion limitations. Pullos compared the limitation of elbow motion with the ULNTT between asymptomatic and neck–arm pain patients. No difference was found between left and right arms in 100 normal subjects. The normal motion deficit was 16.5 to 53.2 degrees with an 8-degree difference between left and right considered normal. In 25 neck–arm pain patients, 68% had positive ULNTT results with asymmetrical elbow motion and symptom provocation. Finally, in a whiplash injury population, Yeung and colleagues examined limitations of knee motion with the slump test (another neurodynamic test) and symptom onset. The addition of knee extension in the slump test increased cervical pain and produced a greater limitation of knee extension.
From the available clinical research, it appears that a positive response is the provocation of the patient’s pain, not to be confused with the normal response in asymptomatic subjects (normal subjects). Additionally, a limitation of motion was present in those studies that compared patients with normal subjects. Interrater reliability is still questionable; therefore, caution should be exercised when comparing the results of two clinicians, even in the same patient population. Although intrarater reliability was reported to be strong, the criteria used was not clearly defined. The specificity and sensitivity have been reported to be high, and recent studies appear to support this concept. The clinician is also wise to continually consider that non-neural structures are being mechanically deformed, which may lead to potential errors in specificity and sensitivity.
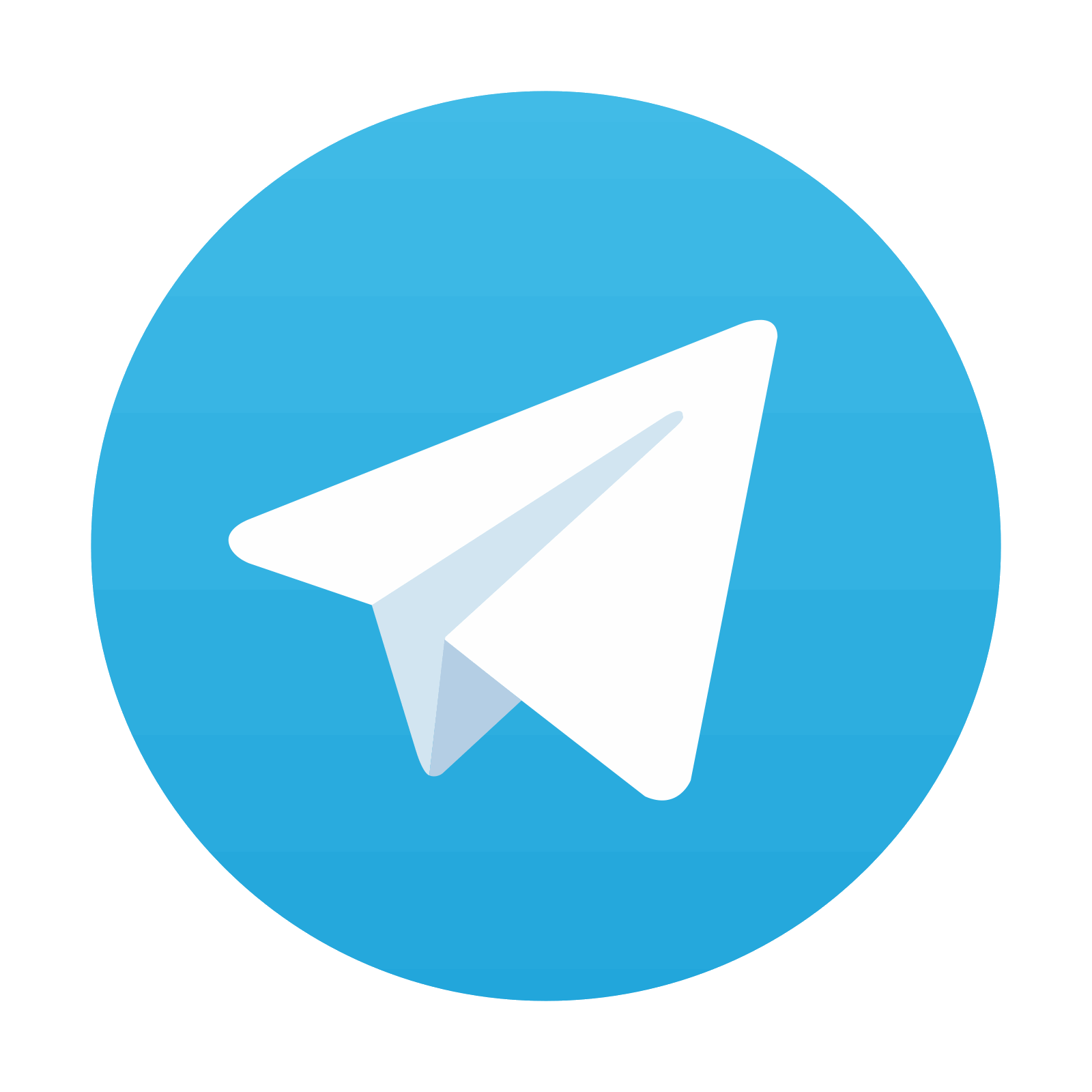
Stay updated, free articles. Join our Telegram channel
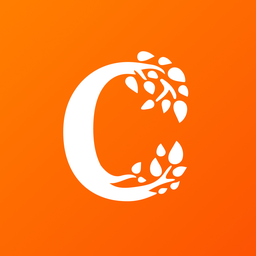
Full access? Get Clinical Tree
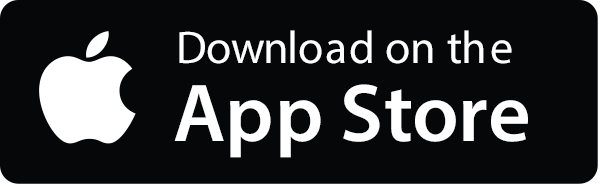
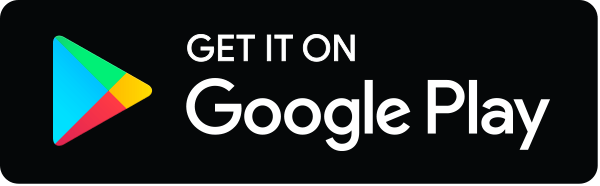