Peripheral nerve injuries are potentially devastating injuries that can lead to profound functional deficits and pain. As peripheral nerves retain the ability to regenerate, understanding the mechanism and physiology of nerve regeneration is critical to improving patient outcomes. Here, we review the physiology of nerve regeneration, as well as review surgical management and new treatment modalities for these injuries.
Introduction
Peripheral nerve injuries (PNI) are prevalent traumatic injuries that can lead to profound sensory, motor and autonomic dysfunction. PNI affects about 2.8% of patients with traumatic injury, accounting for over 5,000 new nerve injuries annually in the United States. Unlike the central nervous system, the peripheral nervous system has the ability to regenerate injured axons, however, this process is complex and time-consuming. Prolonged healing often results in incomplete functional recovery, leaving patients with chronic pain and lasting motor and sensory deficits. In addition to functional deficits, PNI is associated with significant psychosocial and socioeconomic consequences. As our understanding of nerve regeneration and nerve injuries advances, management of PNI continues to progress and has led to improved patient outcomes. Here we review nerve healing, the current surgical management of nerve injuries, advances in nerve regeneration, and emerging novel therapies.
Nerve Injury
PNIs can arise from various mechanisms, most commonly traumatic or compressive injuries. These injuries result in a wide range of functional impairments, depending on the extent and nature of the damage. Nerve injuries are often categorized by the Seddon-Sunderland classification, which describes nerve injuries based on structural injury and prognosis ( Table 1 ). ,
Seddon | Sunderland | Injured | Intact | Recovery Prognosis |
---|---|---|---|---|
Neuropraxia | I | Myelin | Axon | Complete |
Axonotmesis | II | Axon | Endoneurium, perineurium, epineurium | Complete (Slow) |
III | Axon, endoneurium | Perineurium, epineurium | Incomplete | |
IV | Axon, endoneurium, perineurium | Epineurium | Incomplete | |
Neurotmesis | V | Axon, endoneurium, perineurium, epineurium | Poor/None | |
VI | Mixed | Mixed | Mixed |
The Seddon-Sunderland classification describes 5 degrees of nerve injury:
- –
Type I (Neuropraxia): Focal demyelination of the injured axon resulting in a conduction block. Endoneurium, perineurium and epineurium all remain intact. Complete functional recovery is expected.
- –
Type II-IV (Axonotmesis): Progressive damage to the endoneurium and/or perineurium, while the epineurium remains intact. Type II, spontaneous recovery is expected; Type III and IV, recovery is often incomplete and surgical intervention is recommended. ,
- –
Type V (Neurotmesis): Complete nerve transection. Recovery is unexpected without surgical intervention. ,
The Seddon-Sunderland classification has been further expanded to include type VI nerve injuries, or “mixed injury” patterns, representing a varying degree of injuries.
Peripheral Nerve Regeneration
The peripheral nervous system is unique in its ability to regenerate axons that have been impacted by trauma or injury, a process that is not seen in the central nervous system. Following a PNI, a series of coordinated processes occur at both the proximal and distal nerve stumps to prepare the nerve for regeneration.
At the proximal stump, a large influx of calcium and sodium ions into the cytoplasm activates cyclic adenosine monophosphate (cAMP) and initiates chromatolysis, or the dissolution of Nissl bodies. Chromatolysis leads to an increased protein synthesis in preparation for axonal repair and regeneration. Concurrently, cAMP activation promotes the expression and production of cytoskeletal components required for nerve regeneration.
The distal stump undergoes Wallerian degeneration, a crucial process that creates an environment conducive to nerve regeneration and healing ( Fig. 1 ). Similarly, this process is triggered by an influx of calcium ions, along with other cellular signals, ultimately resulting in the disintegration of the axons distal to the injury. During Wallerian degeneration, Schwann cells –specialized glial cells responsible for myelination –dedifferentiate from mature myelinating cells into proliferating “repair” Schwann cells.

Schwann cells play a central role in coordinating the repair process though a cascade of cytokines and chemokines, including IL-6, LIF, and MCP-1, which amplifies the inflammatory response and attracts macrophages to the site of nerve injury. Macrophages subsequently secrete additional cytokines crucial for remyelination. Together, Schwann cells and macrophages clear the cellular debris through phagocytosis, leaving behind a scaffold of an endoneurial basal lamina and elongated immature Schwann cells, called bands of Bungner, which help form a pathway for the regenerating axon to grow.
At the injury site, the proximal nerve end retracts and a connection is made between inflammatory cells to the distal Bungner band, forming a nerve bridge. The nerve bridge upregulates vascular endothelial growth factor (VEGF), cytokines and neurotrophins, creating an environment conducive to axonal growth. As axons slowly regenerate, Schwann cells help guide the sprouting axons to the distal Bungner bands to bridge the nerve gap and then start the process of remyelination. Of note, the new myelin is often thinner and more irregular than in noninjured nerves. ,
On a cellular level, regenerative-associated genes (RAGs) are activated in Schwann cells. , As gene expression changes, Schwann cells downregulate myelin-related proteins and upregulate the expression and secretion of GAP-43, glial cell line-derived neurotrophic factor (GDNF), brain-derived neurotrophic factor (BDNF), and nerve growth factor (NGF). Transcription factor C-Jun is also activated and is a crucial regulator of Schwann cell response to injury. , C-Jun helps reprogram Schwann cells back to a myelinating phenotype and its absence results in impaired regenerative and neuronal death.
Regenerating axons grow in a staggered pattern from the growth cone formed at the proximal stump and progress at a rate of 1-3 mm/day. This slow rate of regeneration poses a significant challenge, as denervated muscles and motor endplates remain viable for only 12-18 months. Beyond this timeframe the irreversible loss of the motor endplates leads to muscular fibrosis and atrophy. , Oxidative stress from the injury further exacerbates muscle atrophy and cell death in target organs limiting recovery potential.
Full spontaneous functional recovery after higher grade PNI is rare, emphasizing the importance of early surgical intervention. Given the inherently slow pace of peripheral nerve regeneration, understanding the cellular and molecular mechanisms underlying this process is critical for developing therapeutic solutions that addresses the limitations.
Surgical Intervention
Nerve injuries pose a significant challenge due to their complex healing processes and time-sensitive nature. Prompt intervention is essential to maximize recovery potential by preserving the viability of motor endplates and minimizing scar formation that may impede regeneration. , After nerve injury, Wallerian degeneration in the distal nerve segments can create scar that impairs axonal growth. ,
The goal of surgical intervention is to prevent or reduce pain in the affected extremity and try to restore lost function. When both distal and proximal stumps are present, direct nerve repair with a tension free coaptation using epineural sutures can be performed and is preferential to other surgical options. Tension-free coaptation is critical, as tension along the nerve has been shown to increase scar formation and negatively impact regeneration and ( Fig. 2 ).

In the setting of a segmental nerve gap or when a primary tension-free repair is not feasible, nerve grafts may be used. Nerve autografts are considered the gold standard for large segmental injuries, such as brachial plexus injuries. , Nerve autograft should be harvested from expendable nerves, such as sural nerve or medial antebrachial cutaneous nerve and donor selection depends on the length of the gap and diameter of the injured nerve. Nerve grafts provide a structurally and biologically compatible scaffold that mediates nerve regeneration; however, donor site morbidity can limit their use. For larger mixed motor and sensory nerves, multiple autografts may be required necessitating the harvest of multiple donor nerves. In these scenarios, a processed, cadaveric, acellularized nerve allograft can be considered. , , Similarly to autograft, acellular nerve allografts serve as a nerve scaffold that mimics the architecture of native neural tissue, which supports nerve growth and angiogenesis. , Although they provide a scaffold for nerve regeneration, they lack functional Schwann cells and neural growth factors like nerve autografts provide.
Although primary epineural nerve coaptation remains the gold standard for nerve repair through precise approximation of outer epineurium; the internal topography and alignment of fascicles is not specifically addresses and hinder functional recovery and outcomes. Nerve dehiscence after primary repair can also occur especially when placed under tension. ,
Overall, the process of neuronal regeneration in peripheral nerves is slow and can be affected by a multitude of factors including, scar, inflammation, ischemia, and a variety of medical comorbidities. As a result, optimizing and improving peripheral nerve regeneration has been widely studied. Here, we discuss therapies that have been utilized or are currently under investigation to improve peripheral nerve regeneration and healing.
Electrical Stimulation
Significant research has focused on optimizing nerve regeneration by improving the rate of axonal growth. Electrical stimulation has emerged as a promising intervention to enhance regeneration across nerve repair sites, with goals of enhancing the regenerative mechanisms of injured nerves and shortening time to recovery. , Electrical stimulation has been widely studied in multiple animal models, which demonstrated accelerated axonal sprouting, faster time to recovery of function and improved nerve regeneration across the repair site. Electrical stimulation upregulates multiple growth-related proteins and increases the production of growth factors, including brain-derived neurotrophic factor (BDNF), glial cell line derived neurotrophic factor (GDNF), and neurotrophin 3. These factors collective promote cell proliferation, migration and adhesion, which are essential for effective nerve healing. In an animal model, electrical stimulation was shown to increase initial number of motor neurons across the nerve repair into distal nerve segment, but did not result in increased axonal growth. Overall, electrical stimulation enhances recruitment of regenerative axons, enhances axonal growth across nerve repair site, and improves preferential selection of axons towards the target. , , ,
Clinically, electrical stimulation has been studied in a variety of nerve conditions, including nerve compression, nerve transections, nerve transfer and preconditioning prior to nerve repair. In the setting of nerve compression, patients who had postoperative electrical stimulation following carpal tunnel release or cubital tunnel release, demonstrated increased axonal regeneration and evidence of increased pinch and grip strength in cubital tunnel patients. , Similar results of accelerated nerve regeneration were seen in patients who suffered digital nerve injury, who were treated with electrical stimulation postoperatively. Electrical stimulation has also been applied in a preventative manner, to reduce risk of shoulder impairment in patients who underwent neck dissection. This study demonstrated patients had improved shoulder function following intra-operative electrical stimulation. ,
Although electrical stimulation demonstrates promising preclinical and clinical findings, the application still remains a challenge as affordable, implantable, commercially-available devices are not yet widely available.
Polyethylene Glycol (PEG) Fusion
Recently, polyethylene glycol (PEG) has emerged as a promising frontier in nerve regeneration. When PEG is mixed with a water-soluble amine solution, like methylene blue, a strong gel substance is formed, which has been demonstrated to have superior strength of repair site when compared to suture repair alone and to decrease perineural scarring. , , , Beyond providing mechanical strength, PEG acts as a fusogen and improves ability of axons to regenerate across the nerve repair site and prevent distal Wallerian degeneration. Clinical outcomes after PEG fusion are currently being investigated in a randomized multi-center study. Its use requires irrigation of the neurorrhaphy site with calcium-free solution, application of methylene blue to freshly trimmed nerve endings, epineural suture repair, and irrigation with a calcium-containing solution ( Fig. 3 ).

PEG fusion requires early intervention, before the onset of Wallerian degeneration, which may limit its utility in the treatment of traumatic nerve injury. However, PEG fusion may improve outcomes after nerve transfer, nerve grafting, or targeted muscle reinnervation. Another limitation of PEG fusion is the nonspecific fusion that occurs which may theoretically prevent restoration of the native anatomy. However, studies have shown that neuroplasticity is able to compensate for “ cross-innervation” and still results in superior clinical outcomes. There is currently limited evidence of PEG in the clinical setting, with only 2 case reports published. , Although limited in sample size, these studies have demonstrated promising results.
Nerve Conduits
Nerve conduits are acellular tubes designed to help bridge small nerve gaps by guiding regenerating axons towards their distal targets. Typically, nerve conduits can be used to bridge gaps up to 30 mm, but studies have shown successful outcomes when used in defects up to 70 mm. , Nerve conduits serve as a bridge between the proximal and distal nerve ends, and helps create a microenvironment conducive to nerve regeneration by preventing infiltration of fibrous tissue, minimizing disorganized axonal sprouting, and reducing glial scar formation.
A variety of materials have been used for nerve conduits, both synthetic and natural materials. Initially, nerve conduits were designed from synthetic material that was relatively nonpermeable and slowly degraded, to allow for fewer sutures and the accumulation of growth factors. Natural materials have also been investigated, including, collagen, chitosan, alginate, and fibrin. With advances in bioengineering, newer synthetic nerve conduits have gained popularity, due to their increased strength and ability to conjugate the conduits with variety of therapeutic agents and hydrogels.
Recently, nerve conduits were investigated as a delivery mechanism for therapeutic agents, stem cells and growth factors. , One example of this is utilizing nerve conduits to locally deliver immunosuppressant tacrolimus (FK506). FK506 has been demonstrated to increase the number of myelinated nerve fibers, the number of regenerating neurons, and improve functional recovery. , As FK506 has side effects if given systemically, a targeted local delivery is superior. In a similar fashion, electrically conductive or self-powered conduits have been investigated. As discussed earlier in this chapter, there is improved neuronal regeneration to electrical stimulation when applied intra-operatively or postoperatively to injured peripheral nerves.
Gene Therapy
Gene therapy has been explored as a strategy to enhance intrinsic regenerative properties of peripheral nerves. The goals of gene therapy are to promote the pro-regenerative phenotype of neuron cell bodies to enhance and sustain axon regeneration, and to maintain pro-regenerative phenotype of distal Schwann cells to provide a receptive environment for axon regrowth into distal nerve stump. Adeno-associated viral (AAV) vectors are the most commonly used delivery mechanism for gene therapy. These vectors have been utilized to deliver genes such as GDNF and vascular endothelial growth factor (VEGF), which have shown neuroprotective effects and enhanced axonal growth in animal studies. , Additionally, gene therapy targeting Schwann cells has demonstrated significant promise. The transcription factor c-Jun promotes a pro-regenerative state in Schwann cells following nerve injury. and increased c-Jun expression has been shown to reprogram Schwann cells, preventing the loss of their regenerative capacity and sustaining axonal regrowth.
Another target of gene therapy is sterile alpha receptor motif containing 1 (SARM-1), which has been identified as a key protein that triggers Wallerian degeneration. Following nerve injury, the influx of calcium (Ca ++ ) depletes adenosine triphosphate (ATP). As a result, the mitochondrial cell membrane is destabilized and further increases in Ca ++ initiates Wallerian degeneration. Within hours of injury, the distal nerve stump begins to be broken down and cleared by macrophages. Animal models have shown that blocking SARM-1 prevents calcium influx and Wallerian degeneration, thereby extending the window for axonal regeneration. , In zebrafish, SARM-1 deficient axons remain viable for 5 days after injury, significantly longer than wild type axons that degraded in 8 hours. , As Wallerian degeneration takes place within hours of nerve injury, the opportunity and application of SARM-1 inhibitors needs to be investigated.
Although gene therapy has shown promising results in animal models, further translational and clinical studies are required to determine their effectiveness as well as determining best delivery methods.
Conclusion
Peripheral nerve injury can result in functional deficits that often necessitates surgical intervention. Understanding the physiology of nerve regeneration and healing is critical to optimizing nerve repair and regeneration. As technology advances, nerve healing can be enhanced using multiple modalities and interventions including electrical stimulation, PEG fusion, and gene therapy. Though these interventions have demonstrated promising results, further research is required to bring these interventions into a clinical setting.
Declaration of Interest Statement
The authors report no potential conflicts of interest.
Funding: No grant funding or other financial support was provided for this chapter.
References
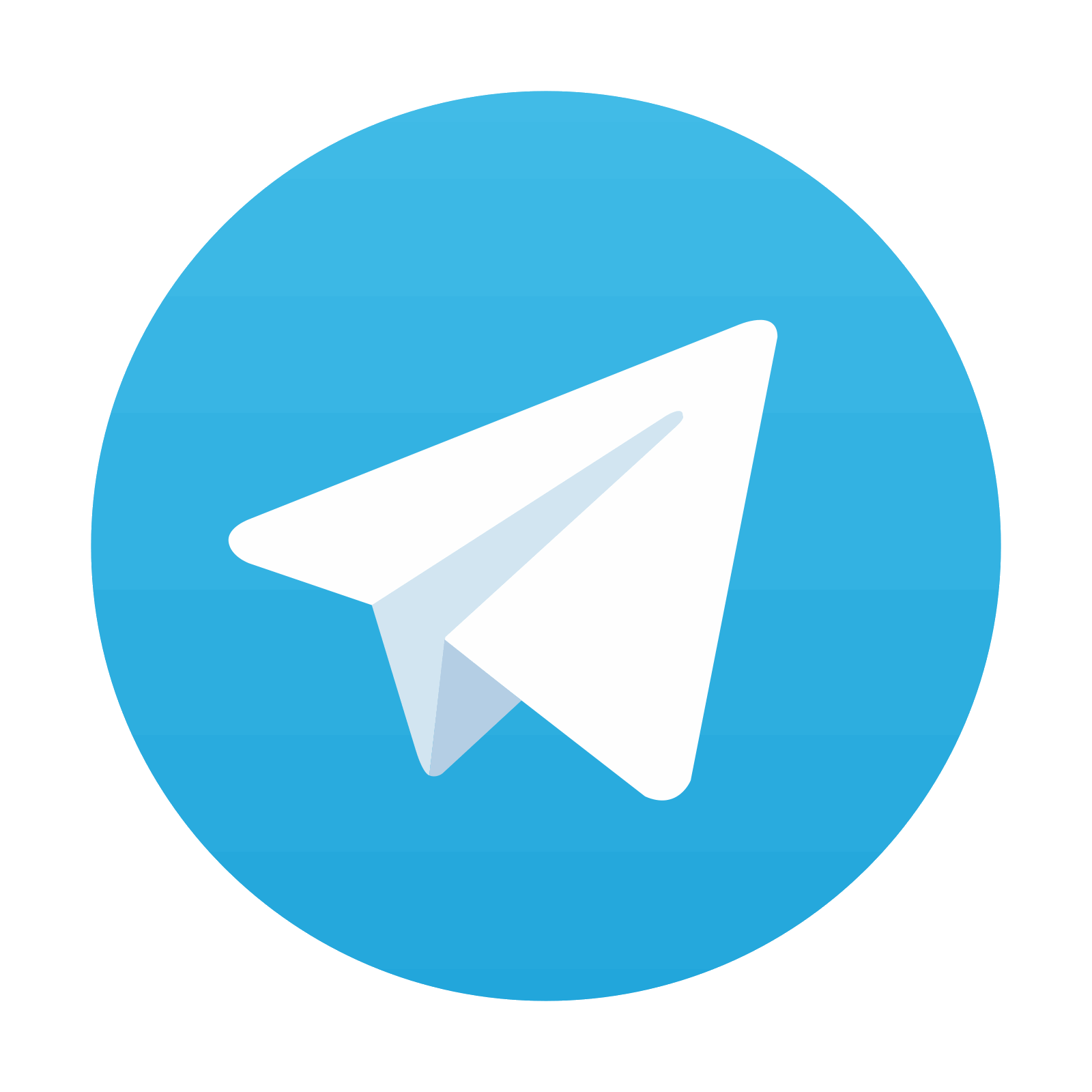
Stay updated, free articles. Join our Telegram channel
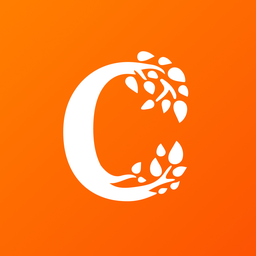
Full access? Get Clinical Tree
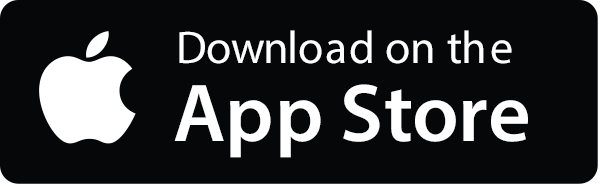
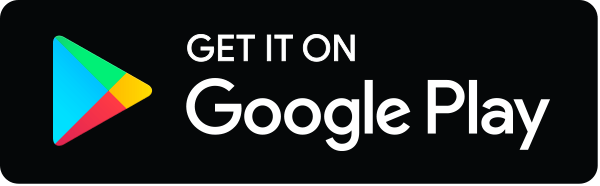
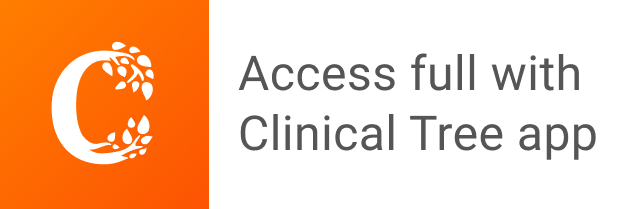