Pearls
- •
Receptors play a central role in determining the nature of the pharmacologic effects produced by a drug.
- •
Most drugs and endogenous compounds (e.g., hormones, neurotransmitters) exert their action by binding to a receptor or by modulating an ion channel.
- •
G proteins are a superfamily of proteins that allow transduction between an activated receptor (by an agonist) and different intracellular effectors, such as enzymes or ion channels, relaying signals from more than 1000 receptors. G protein–coupled receptors are complex signaling machines that participate in most physiologic and pathophysiologic processes and represent the target, directly or indirectly, of approximately 40% of all current therapeutic agents.
- •
Continued exposure of a receptor to an agonist often results in progressive loss of receptor responsiveness, with a diminished receptor-mediated response over time. This is called desensitization .
- •
Two main superfamilies of membrane transporters provide the active transport of drugs to their target receptors in specific organs and tissues: the adenosine triphosphate–binding cassette superfamily and the solute carrier family.
- •
Calcium is critically important as a regulator of cell function. It exerts its control on cellular function through its ability to regulate the activity of many different proteins, such as channels, transporters, and transcription factors.
- •
An individual’s genetic makeup can modify the efficacy of drug treatment and the risk of adverse reactions.
Optimizing drug response is a challenging task that clinicians confront on a daily basis. This is particularly true for those caring for critically ill patients, in whom many factors influencing drug response are being more commonly recognized. These include reduced absorption, variable drug distribution, decreased metabolism and elimination, as well as alterations in drug receptors, signaling mechanisms, and effectors. Advances in molecular pharmacology have shed more light on the processes that transduce extracellular signals into intracellular messages that control cell function. This has led to the elucidation of multiple points at which modulation of signal transduction, by either pharmacologic agents or diseases, can occur. Also, there has been an ongoing recognition of the role of inheritance in the individual variation of drug response with the identification of polymorphisms in genes encoding drug-metabolizing enzymes, drug targets (e.g., receptors, enzymes), and proteins involved in signal transduction.
This chapter provides an overview of how drugs work at the molecular level and how this complex system is influenced by genetic factors, developmental changes, disease processes, and the environment ( Fig. 123.1 ). Ultimately, the objective is to help pediatric intensive care providers better tailor the pharmacotherapy that they use—to choose the right drug, or combination of drugs, for the right patient to achieve maximal efficacy with the least toxicity. This chapter does not address signaling pathways involved in diseases per se.

Targets for drug action
The initial step in the cascade of biochemical events resulting in drug action mostly consists in the binding of drugs to specific cellular targets. These can be broadly divided into four categories: (1) receptors, (2) ion channels, (3) enzymes, and (4) carrier proteins ( Fig. 123.2 ). The majority of important drugs act on one of these types of proteins. Table 123.1 shows the targets of some pharmacologic agents commonly used in the pediatric intensive care unit (ICU).

Drug | Target | Agonist | Antagonist |
---|---|---|---|
Receptors | |||
G Protein–Coupled Receptors | |||
Adenosine | Adenosine | ✓ | |
Atropine | Muscarinic | ✓ | |
Bosentan | ET A , ET B | ✓ | |
Clonidine | α 2 -Adrenergic | ✓ | |
Dopamine | D 1 | ✓ | |
Dopamine | α- and β-Adrenergic | ✓ | |
Dobutamine | β-adrenergic | ✓ | |
Epinephrine | α- and β-Adrenergic | ✓ | |
Haloperidol | D 2 | ✓ | |
Isoproterenol | β-Adrenergic | ✓ | |
Neuromuscular blockers (depolarizing and nondepolarizing) | Nicotinic | ✓ | |
Norepinephrine | α- and β-Adrenergic | ✓ | |
Opioids | μ, δ, κ opioid | ✓ | ✓ |
Phenoxybenzamine | α-Adrenergic | ✓ | |
Phenylephrine | α 1 -Adrenergic | ✓ | |
Propranolol | β-Adrenergic | ✓ | |
Ranitidine | H 2 | ✓ | |
Vasopressin | V 1 , V 2 , V 3 | ✓ | |
Salbutamol | β 2 -Adrenergic | ✓ | |
Channel-Linked Receptors | |||
Barbiturates | GABA A -gated Cl − | ✓ | |
Benzodiazepines | GABA A -gated Cl − | ✓ | |
Flumazenil | GABA A -gated Cl − | ✓ | |
Ketamine | Glutamate-gated (NMDA) cation | ✓ | |
Enzyme-Linked Receptors | |||
Nitric oxide | Soluble guanylate cyclase | ✓ | |
Insulin | Insulin | ✓ | |
Nuclear Receptors | |||
Glucocorticoids | Glucocorticoid | ✓ | |
Spironolactone | Mineralocorticoid | ✓ | |
Ion Channels | |||
Adenosine | Ca 2 + | ✓ | |
Amiodarone | Na + , K + , Ca 2+ | ✓ | |
Lidocaine | Na + | ✓ | |
Enzymes | |||
Acetazolamide | Carbonic anhydrase | ✓ | |
Captopril | Angiotensin-converting enzyme (peptidyl dipeptidase) | ✓ | |
Milrinone | Phosphodiesterase III | ✓ | |
Nonsteroidal antiinflammatory drugs | Cyclooxygenase-1 and -2 | ✓ | |
Sildenafil | Phosphodiesterase V | ✓ | |
Ion Pumps and Transporters | |||
Digoxin | Na + /K + -ATPase pump | ✓ | |
Loops diuretics | Na + /K + /Cl − cotransporter | ✓ | |
Omeprazole | H + /K + -ATPase pump | ✓ | |
Thiazides | Na + /Cl − cotransporter | ✓ |
Receptors
Receptors are the most frequent drug target. They can be defined as the sensing elements in the system of chemical communication that coordinate the function of all of the different cells in the body, the chemical messengers being the various hormones, neurotransmitters, other mediators, or drugs. Clark’s theory of receptor occupancy posits that the drug effect is directly proportional to the number of occupied receptors. While not always true, this concept is useful in illustrating the concepts of selectivity, affinity, and efficacy ( Fig 123.3 A). Selectivity refers to the preference of a drug for one receptor over another ( Fig 123.3 B). A perfect drug binds only to its target receptor. In reality, drugs also bind to other receptors and can result in off-target “side” effects. Even drugs with very high selectivity can result in off-target effects if they bind to their target receptor in a nontarget organ. Affinity refers to the strength of the drug-receptor interaction. Efficacy is the ability of a drug to elicit the desired response and is generally represented by a sigmoid concentration-effect curve. The shape of this curve is a function of selectivity, affinity, and efficacy and reflects a drug’s potency. The concentration at which half of the maximal effect is attained is the EC50. A smaller EC50 means that less drug is needed to achieve the same effect.

Drugs can be further classified based on their concentration-effect relationship. Drugs that bind with receptors are termed agonists if their binding results in the expected effect and are termed antagonists if binding stops or decreases an agonist-induced activity ( Fig. 123.3 C). Administration of a receptor antagonist in the absence of an agonist results in no effect because antagonists bind to receptors but do not activate them. Competitive antagonism (surmountable or reversible) is when a drug with affinity for a receptor but lacking intrinsic efficacy competes with the agonist for the primary binding site on the receptor. In such an antagonism, there is a concentration-dependent production of a parallel shift to the right of the agonist dose-response curve with no change in the maximal response ( Fig 123.3 C). In the case of noncompetitive antagonism (insurmountable or irreversible) with a slowly dissociating or nondissociating antagonist, there is a shift of the dose-response curve to the right and a further depression of the maximal response; no change in the antagonist occupancy takes place when the agonist is applied ( Fig. 123.3 C).
The duration of action of an insurmountable antagonist depends mostly on synthesis of new receptors, which can take several days. This may have clinically important consequences. For example, phenoxybenzamine, an insurmountable α-adrenergic receptor antagonist sometimes used in stage I Norwood procedures to balance the pulmonary and systemic circulations, can produce symptomatic hypotension in some patients. The attenuation or reversal of the decrease in systemic vascular resistance that it produces may not be achieved with an α-adrenergic receptor agonist such as dopamine or norepinephrine, depending on the dose of phenoxybenzamine given. In such circumstances, the use of a pressor agent that does not act through the α-adrenergic receptor—such as vasopressin, which binds on V 1 receptors of smooth muscle cells—must be considered.
Partial agonists are ligands that bind to the same receptor as full agonists but have less intrinsic capacity to produce a response as strong as full agonists despite full receptor occupancy ( Fig. 123.3 C). The exact mechanism that accounts for the blunted maximal response seen with partial agonists is unknown. Simultaneous administration of a partial agonist and a full agonist prevents the maximal response usually observed with the full agonist alone because partial agonists have the ability to occupy the receptor population. Consider what would happen to the maximum effect (E max ) of an agonist in the presence of increasing concentrations of a partial agonist. As the number of receptors occupied by the partial agonist increases, the E max would decrease until it reached the E max of the partial agonist. This potential of partial agonists to act both agonistically and antagonistically may be therapeutically exploited. For example, aripiprazole, an atypical neuroleptic agent, is a partial agonist at selected dopamine receptors. Dopaminergic pathways that were overactive would tend to be inhibited by the partial agonist, whereas pathways that were underactive may be stimulated. This might explain the ability of aripiprazole to improve many of the symptoms of schizophrenia, with a small risk of causing extrapyramidal adverse effects. Finally, inverse agonists are ligands that reduce the level of constitutive activation encountered in some receptor systems.
In addition to the primary binding site to which agonists and antagonists bind, receptor proteins possess many other (allosteric) binding sites through which drugs can influence receptor function by increasing (allosteric facilitators) or decreasing (allosteric antagonists) the affinity of agonists for their binding site, or by modifying efficacy. The resulting effect may be to alter the slope and maximum of the agonist concentration-effect curve. An example of allosteric facilitation includes the activation of benzodiazepines on γ-aminobutyric acid A (GABA A ) receptors.
In summary, receptors play a central role in determining the nature of the pharmacologic effects that a drug produces. First, receptors bind with only one or a limited number of structurally related ligands, thus ensuring that the final effect seen in a normal setting occurs only in response to defined stimuli. Second, for a given dose or concentration of a drug, both that drug’s affinity to bind to the receptor and the total number of available receptors directly influence the maximal effect a drug can produce. Third, drugs differ in their intrinsic activity in regard to their receptors (e.g., partial agonist versus full agonist). Thus, the magnitude of the response to any drug is proportional to both the extent of receptor occupancy and the intrinsic activity of the receptor itself, resulting in different dose or concentration relationships for different agonists.
Ion channels
Ion channels are molecular machines that serve as principal integrating and regulatory devices for the control of cellular excitability. Different types of ion channels have been described: channels responding to electrical (voltage-dependent ion channels), mechanical, or chemical (ligand-gated ion channels) stimuli; ion channels controlled by phosphorylation/dephosphorylation mechanisms; and G protein–gated ion channels. Most ion channels are of the voltage-dependent type and consist mainly of sodium (Na + ), potassium (K + ), and calcium (Ca 2+ ) channels. Drugs can affect ion channel function directly by binding to the channel protein and altering its function or indirectly through G proteins and other intermediates. Lidocaine is a good example of a drug that directly affects voltage-gated Na + channels by blocking the channel and thus Na + entry into the cell. Channel-linked receptors (ligand-gated ion channels) are discussed later.
Enzymes
Enzymes are a specialized class of proteins responsible for catalyzing chemical reactions within the cell and thus are ideal drug targets. Most drugs that alter enzyme activity are substrate analogs of enzymes that inhibit their activity either reversibly (e.g., angiotensin-converting enzyme inhibitors acting on peptidyl dipeptidase) or irreversibly (e.g., acetylsalicylic acid acting on cyclooxygenase). Drugs may also prevent the normal functioning of enzymes. Fluorouracil, an anticancer drug, is a good example; it is converted into an abnormal nucleotide that inhibits thymidylate synthetase thus blocking DNA synthesis.
Carrier proteins
Several biological elements, such as ions and small organic molecules, are not lipid soluble enough to cross membranes and require a carrier protein (an ion pump or a membrane transporter) to do so. Some carrier proteins use the energy of adenosine triphosphate (ATP) hydrolysis to move ions or small molecules across a membrane against a chemical concentration gradient or an electrical potential (primary active transport; Fig. 123.4 ). These include ion pumps (or ATPases), which transport ions only, and the ATP-binding cassette (ABC) transporters, which catalyze transmembrane movements of various organic compounds, including amphipathic lipids and drugs. Other carrier proteins, such as transporters from the solute carrier (SLC) superfamily, use energy derived secondarily from the electrochemical potential generated by the concentration gradient of sodium or potassium under the control of the Na + /K + -ATPase pump itself coupled with ATP hydrolysis (secondary active transport; see Fig. 123.4 ). In such instances, the transport of organic molecules is usually coupled to the transport of hydrogen (H + ) or Na + , either in the same direction (symport) or opposite direction (antiport). The SLC membrane transporters include more than 380 members organized into 52 families. Solute carrier transporters facilitate the transport of a wide array of substrates across biological membranes and have important roles in physiologic processes ranging from the cellular uptake of nutrients to the absorption of drugs and other xenobiotics. The Na + /H + exchanger (NHE), Na + /Ca 2+ exchanger (NCX), and Na + /K + /Cl – cotransporters belong to this superfamily.

The carrier proteins embody a recognition site that makes them specific for a particular permeating species; these recognition sites can also be targets for drugs whose effect is to block the transport system (see Fig. 123.2 ). Indeed, some drugs bind to these carrier proteins and interfere with the transport system. Digoxin is a typical example of drugs that produce their effect by blockade of an ion pump (the Na + /K + -ATPase pump), while furosemide is a typical example of drugs that block a membrane transporter (the Na + /K + /Cl – cotransporter).
Transporters are not just potential drug targets—they play an important role in allowing drugs to reach their site of action in specific organs and tissues. To reach its target, a drug may have to cross several membranes. For example, an oral drug that is active in the brain needs first to cross intestinal epithelial cells and then the blood-brain barrier. The majority of drugs cross cell membranes using an active transport mechanism, either primary or secondary (as discussed previously), that is under the control of membrane transporters. Membrane transporters are widely expressed throughout the body, most notably in the epithelia of major organs, such as the liver, intestine, kidney, and organs with barrier functions, such as the brain, testes, and placenta.
Several membrane transporters have been discovered. The ABC and the SLC superfamilies are the two main types of membrane transporters involved in drug transport. The ABC superfamily has more than 50 members distributed in different families with those mainly involved in the transport of drugs consisting of the multidrug resistance (MDR) family, the multidrug resistance protein (MRP) family, the breast cancer resistance protein, and the bile-salt export protein. The main function of these ABC transporters is to facilitate the efflux of drugs from the cells through apical and basal membranes. P-glycoprotein (PgP) or MDR1, the most extensively studied ABC transporter, is widely distributed with a broad substrate specificity (e.g., tacrolimus, digoxin, dexamethasone, chemotherapeutic agents [etoposide, doxorubicin, vinblastine], protease inhibitors) and is subject to drug interactions through inhibition or induction. It limits intestinal drug absorption, participates in drug elimination (liver and kidney), and influences drug tissue distribution (e.g., it limits drug entry into the brain). Some cancer cells also express large amounts of PgP, rendering these cancers multidrug resistant.
Membrane transporters of the SLC superfamily mainly involved in the transport of drugs consist of organic anion transporting polypeptide, organic cation transporter, organic cation transporters novel, organic anion transporter (OAT), organic anion/urate transporter, multidrug and toxin extrusion protein, heteromeric organic solute transporter, peptide transporter, humane equilibrative nucleoside transporter, Na + -dependent taurocholate cotransporting polypeptide, and monocarboxylate transporters. The main function of the SLC family is to facilitate the entry and the elimination of drugs from the cells through apical and basal membranes.
Receptor type and regulation
Four families of receptors, three cell surface receptor types and one nuclear receptor, have been described ( Fig. 123.5 ). Most transmembrane signaling is accomplished by only a few molecular mechanisms, each of which has been adapted to transduce many different signals. These protein families include cell surface receptors and receptors within the cell as well as enzymes and other components that generate, amplify, coordinate, and terminate post-receptor signaling.

G protein–coupled receptors
In 1994, Alfred G. Gilman and Martin Rodbell were awarded the Nobel Prize in physiology or medicine for their discovery of G proteins and their role in signal transduction in cells. Furthermore, in 2012, Robert Lefkowitz and Brian Kobilka were awarded the Nobel Prize in chemistry for their discoveries that reveal the inner workings of the β-adrenergic receptor, which led to the seminal discovery that all G protein–coupled receptors (GPCRs) have a similar molecular structure. G proteins are a superfamily of propeller proteins that allow the transduction between the activated receptor (by an agonist) and different intracellular effectors such as enzymes or ion channels, relaying signals from more than 1000 receptors. GPCRs, also known as metabotropic receptors , are the first component of the cellular amplification cascade ( Fig. 123.6 ). The activation of target enzymes through GPCRs leads to the synthesis of numerous second messengers, which, in turn, activate other enzymes. The intervention of the second messenger system allows for the diversity of the cellular targets (discussed later). GPCRs are complex signaling machines that participate in most physiologic and pathophysiologic processes and represent the target, directly or indirectly, of approximately 40% of all current therapeutic agents. Of note, pharmacologic agents have been developed for approximately only 10% of GPCRs so far.

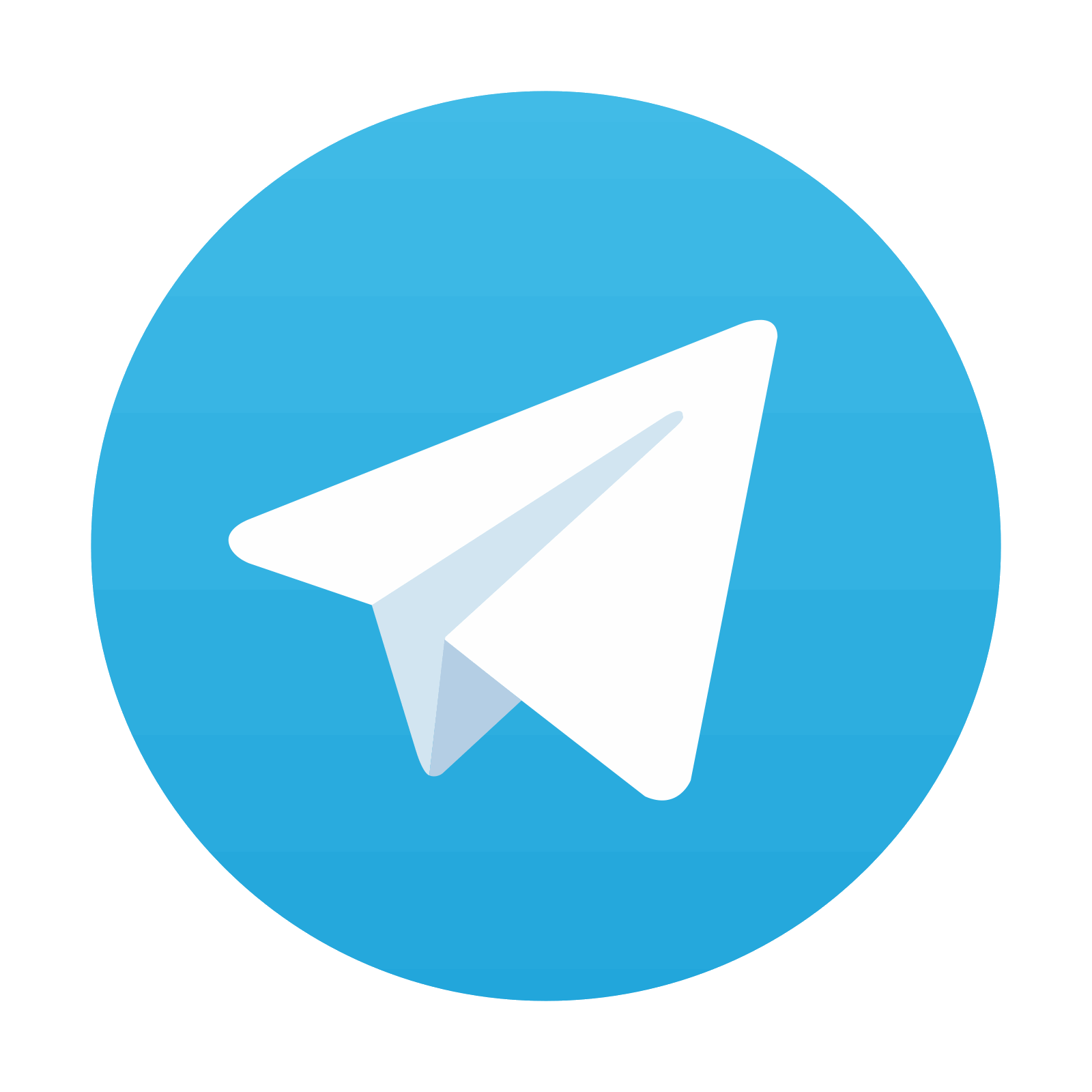
Stay updated, free articles. Join our Telegram channel
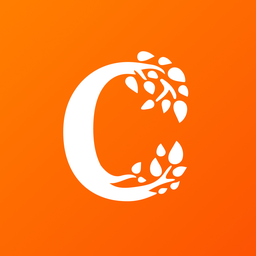
Full access? Get Clinical Tree
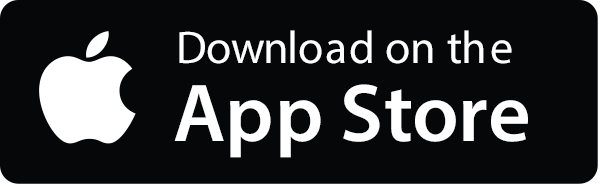
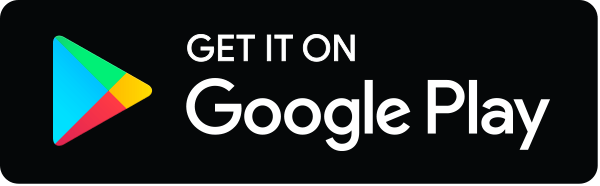
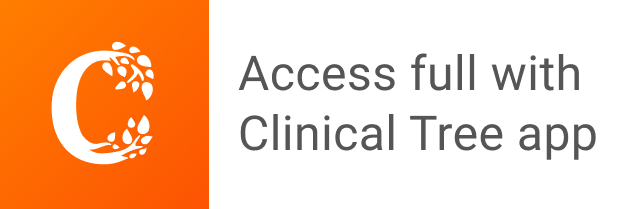