Skeletal muscles not only use glycogen but also lipid as a source of energy. The oxidation of lipids occurs in the mitochondria and involves a series of enzymatic reactions ( Fig. 18.1a, b ). Defects in these pathways and in the pathways of oxidative phosphorylation in the mitochondria lead to a heterogeneous group of muscle disorders.


Disorders of Muscle Lipid Metabolism
The first association of a myopathy with a disorder of lipid metabolism was the report by , who suggested the possibility in 18-year-old identical twin sisters, who from childhood had muscle cramps associated with myoglobinuria, at times occurring some hours after exercise. Carbohydrate metabolism was normal. Attacks could be provoked by prolonged fasting or by a high-fat, low-carbohydrate diet. Muscle biopsy was histologically normal but showed excess lipid droplets on oil red O staining. They postulated a defect in the utilization of long-chain fatty acids. In a prophetic annotation in the same journal, predicted a deficiency of either carnitine or carnitine palmitoyl transferase (CPT) to account for this lipid storage myopathy. were subsequently able to demonstrate carnitine deficiency in the muscle of a 24-year-old woman with weakness all her life and progression from the age of 19 years. Muscle biopsy showed a vacuolar-like myopathy filled with lipid droplets on histochemical staining. In the same year, reported a 29-year-old man with episodic cramps and myoglobinuria of 16 years’ duration but no muscle weakness. Muscle biopsy showed no excess of lipid but a deficiency of the enzyme CPT.
Morphological Aspects
The triglycerides are stored in muscle in the form of lipid droplets ( ). Under the light microscope they are readily revealed by stains for neutral fat such as oil red O or Sudan black. The droplets are more numerous in the type 1 fibres and tend to be concentrated more at the periphery of the fibre. The presence of excess lipid can give a very vacuolated-like appearance with routine histological stains such as haematoxylin and eosin (H&E) and may be more marked in type 1 fibres ( Figs. 18.2–18.4 ), but as lipid droplets are not membrane bound they are not vacuoles. Under the electron microscope, the lipid droplets appear as empty, rounded spaces of uniform size and do not have a limiting membrane. They are located between the myofibrils and under the sarcolemma and are often adjacent to mitochondria. An excess of lipid, as in carnitine deficiency, shows as a striking increase in the number and size of the droplets under light microscopy, as well as with electron microscopy, and may be associated with structural abnormalities in the mitochondria (see Fig. 18.4 ). In cases with CPT II deficiency, however, the amount of lipid may not appear particularly increased, and in other enzyme defects it varies with the metabolic state of the patient.



The primary lipid metabolism disorders with most extensive lipid storage are neutral lipid storage disease, lipin-1 deficiency, systemic (primary) carnitine deficiency and multiple acyl-CoA dehydrogenase deficiency. However, mitochondrial diseases (see below) frequently show lipid storage secondary to defective oxidative phosphorylation. Other conditions that may also show lipid storage include inflammatory and drug-induced myopathies. Congenital myasthenic syndromes associated with DOK7 mutations were also reported to be associated with lipid accumulation in several cases ( ).
Clinical Syndromes Associated with Abnormalities in Fatty Acid Metabolism
The clinical features of lipid disorders of muscle fall into two broad groups: those in which muscle symptoms are the predominant abnormality and those in which muscle involvement is part of a more general systemic illness ( ). In patients in whom the muscle involvement is the major or only clinical feature, the presenting symptoms and signs may be proximal or diffuse muscle weakness, or muscle pain, particularly on prolonged exertion, which may be associated with muscle necrosis and myoglobinuria. The muscle symptoms seen in children in whom muscle involvement is part of a systemic illness are predominantly hypotonia and generalized muscle weakness. In some, the symptoms may resolve as the clinical condition improves; in others, the hypotonia and muscle weakness persist, and recovery may take several months.
Neutral Lipid Storage Disease
Neutral lipid storage disease with myopathy may present in childhood or adolescence as slowly progressive muscle weakness. The muscle weakness is usually proximal but may be predominantly distal. Predominantly affected muscles are found in the posterior compartments of the legs, in the shoulders and upper arms and in paraspinal muscles ( ). The disease is sometimes accompanied by cardiomyopathy ( ). Muscle biopsy shows massive lipid accumulation and rimmed vacuoles are frequently encountered (see Fig. 18.2 ). Lipid droplets are also present in leucocytes. Mutations are found in the PNPLA2 gene, which encodes adipose triglyceride lipase (ATGL), which catalyzes the first step in hydrolysis of triacylglycerol ( ) (see Fig. 18.1a ).
Neutral lipid storage disease with ichthyosis is a childhood-onset disease with congenital ichthyosiform erythroderma, mild myopathy and hepatomegaly. Several other manifestations may be present, including mental retardation, microcephaly, hearing loss and cataracts. There is lipid accumulation in various cell types, including muscle fibres and blood leucocytes. Mutations have been identified in ABHD5 encoding the protein CGI-58, which is an activator of ATGL ( ).
Lipin-1 Deficiency
Lipin-1, encoded by the LPIN1 gene, converts phosphatidic acid to diacylglycerol, which is an important step in triglyceride and phospholipid synthesis. Mutations in LPIN1 have been identified as a cause of severe and occasionally lethal recurrent rhabdomyolysis in children ( ). Muscle histology is either normal or shows moderate abnormalities, including lipid accumulation, predominance of type 1 muscle fibres, atrophy of type 2 fibres and, rarely, subsarcolemmal aggregates of mitochondria (see Fig. 18.3 ). If biopsy is performed shortly after an episode of rhabdomyolysis, necrotic fibres and regeneration may be apparent.
Carnitine Deficiency
Carnitine (β-hydroxy-α-trimethylaminobutyric acid) is the indispensable carrier of medium- and long-chain fatty acids across the inner mitochondrial membrane into the mitochondrion, where they undergo β-oxidation. There are two sources of carnitine – diet and synthesis. The synthesis of carnitine (which is dependent on two essential amino acids, lysine and methionine) takes place predominantly in the liver, and carnitine is then transported by the blood to other tissues. The highest concentration of free carnitine is in muscle, followed by liver, with about half the concentration, and the heart, which is lower still. Carnitine is transported across the cell membrane in many tissues by means of the high-affinity plasma membrane sodium-dependent carnitine transporter (OCTN2).
The selective excretion of acylcarnitine provides a route for removal of accumulating intermediates in various metabolic disorders. Excessive loss of these specific acylcarnitines in the urine results in loss of free carnitine and increases the probability of secondary carnitine deficiency. Such deficiency is seen in various disorders of β-oxidation and oxidative phosphorylation in the mitochondria and secondary to various drugs ( ).
Systemic Carnitine Deficiency
Systemic (primary) carnitine deficiency is caused by mutations in the carnitine transporter OCTN2 encoded by the SLC22A5 gene ( ). In addition to the lipid storage myopathy and cardiomyopathy that usually start in childhood, these patients may also have recurrent episodes of acute hepatic encephalopathy, with nausea, vomiting, confusion or coma (reminiscent of Reye syndrome) and, in some, associated hypoglycaemia, and a metabolic acidosis caused by increased levels of lactate and ketoacids.
Of the eight cases reviewed by , six had died from cardiorespiratory failure, five of them before 20 years of age. In two patients, the weakness worsened towards the end of pregnancy or after delivery. The serum carnitine concentration was markedly reduced in all patients tested. The serum creatine kinase (CK) was elevated in some but not in others and electromyography showed a myopathic pattern.
Replacement therapy with oral carnitine is feasible in systemic carnitine deficiency ( ) as well as in secondary carnitine deficiency ( ).
Muscle biopsy shows severe lipid storage and there may also be mitochondrial proliferation and mitochondrial ultrastructural abnormalities (see Fig. 18.4 ).
Carnitine Palmitoyl Transferase Deficiency
Carnitine palmitoyl transferase is an enzyme that catalyzes the reversible reaction of carnitine and long-chain fatty acyl groups. It exists in two forms, CPT I and CPT II. CPT I is located on the inner side of the outer mitochondrial membrane, whereas CPT II is on the inner side of the inner mitochondrial membrane. Deficiency of either CPT I or CPT II can present as attacks of hypoketotic hypoglycaemia, but the most common manifestation of CPT II deficiency is the benign myopathic form ( ).
Myopathy Associated with CPT II Deficiency
The adult myopathic form of CPT II deficiency is characterized by recurrent myoglobinuria, often precipitated by prolonged exercise, fasting or a combination of the two. reviewed 16 cases from the literature and added three of their own, plus two others in which they had studied the biopsies. Most patients recalled recurrent muscle pains since childhood but they did not seem to have cramps during exercise, which act as warning signals in patients with phosphorylase or phosphofructokinase (PFK) deficiency to stop using their muscles. This may explain the earlier onset as well as the greater frequency of myoglobinuria in CPT deficiency than in phosphorylase or PFK deficiency. By the time patients with CPT deficiency experienced muscle stiffness or pain, it was usually too late to avoid myoglobinuria. The myoglobinuria usually followed vigorous exercise of a few hours’ duration, such as a long hike or a football game, and fasting before exercise was recognized by most patients as a precipitating factor. Five of the 21 had renal failure. In about one-third of cases, there was no apparent precipitating cause for at least some of the episodes of myoglobinuria.
During attacks, affected muscles became swollen, tender and weak. Respiratory muscles were often severely involved, and three cases needed assisted ventilation. Between attacks, patients were usually normal and did not show residual weakness. In 19 of the 21 cases the diagnosis was established between 15 and 30 years of age.
Serum CK was normal between attacks, and blood lactate rose normally after ischaemic exercise. Plasma lipids were abnormal in four cases, with increased triglycerides and cholesterol in three and slightly increased triglycerides in one.
Muscle biopsies taken during the quiescent phase between attacks were normal in about two-thirds of the reported cases. When lipid storage was present, this was usually less marked than in carnitine deficiency, and in two reports was only noted in one out of two biopsies on the patients. Areas of necrosis noted in the limb muscle of one patient and the intercostal muscle of another were probably related to a recent episode of myoglobinuria. Liver biopsy in one case showed no lipid storage but some abnormality of mitochondria, and the morphology of leucocytes was normal in two cases. In general, muscle biopsies rarely show significant pathological changes in CPT deficiencies and levels of lipid within fibres often appear normal. If a sample is taken soon after an episode of myoglobinuria, however, necrosis and fibre regeneration will be apparent.
The diagnosis of CPT II deficiency should be considered in any patient with recurrent myoglobinuria, particularly if precipitated by prolonged exercise or fasting. Two clinical criteria are helpful in distinguishing it from phosphorylase or PFK deficiency: (1) there is no intolerance to vigorous exercise of short duration and no ‘second-wind’ phenomenon, and (2) cramps are unusual and contracture is not induced by ischaemic exercise. Long-chain acylcarnitines may be increased in blood (C 16 , C 18:1 , C 18 ), and the CPT II activity can be measured in fibroblasts, muscle tissue or leucocytes. Mutations are found in the CPT2 gene ( ).
Acyl-CoA Dehydrogenase Deficiency
Before the β-oxidation of fatty acids can occur, they have to be converted first to their coenzyme A (CoA) thioesters, which is catalyzed by the acyl-CoA synthetases in cytosol. The fatty acyl-CoA is then reacted with carnitine by CPT I and transported into the mitochondria by carnitine-acylcarnitine translocase. Carnitine is removed by CPT II and fatty acyl-CoA then enters the β-oxidation pathway, where the initial step is oxidation by acyl-CoA dehydrogenases. There are several types of acyl-CoA dehydrogenases (see Fig. 18.1b ). Defects of the acyl-CoA dehydrogenases are the most frequently identified abnormalities of fatty acid oxidation.
Short-Chain Acyl-CoA Dehydrogenase (SCAD) Deficiency
This deficiency has been described in different clinical situations ( ). There is a myopathic form in which the defect is limited to muscle and presents with a slowly progressive muscle weakness and exercise-induced pain ( ) and a systemic form with hepatomegaly and microcephaly ( ). A 16-year-old girl documented by had recurrent myoglobinuria, hypoketotic hypoglycaemia, encephalopathy and an associated cardiomyopathy. Muscle histology may in some cases show lipid storage and also multicore changes with staining for oxidative enzymes ( ). The presence of increased ethylmalonic acid in urine is a major biochemical marker. SCAD deficiency is associated with recessive mutations in the ACADS gene ( ).
Medium-Chain Acyl-CoA Dehydrogenase (MCAD) Deficiency
This may be one of the most commonly inherited metabolic disorders, with an incidence of 1 in 5000 to 10,000 live births. It usually presents in infancy with an episodic illness in which muscle symptoms and signs are not prominent ( ). The clinical presentations include sudden infant death, Reye syndrome and hypoglycaemic episodes. Some cases, however, present in later life, and exercise-induced muscle pain may be a feature; yet others are asymptomatic and are detected only when the disorder is diagnosed in another family member ( ). MCAD deficiency is inherited in an autosomal recessive pattern and is associated with mutations in the ACDM gene ( ). Increased amounts of medium-chain acylcarnitines are present in blood and urine.
Very Long-Chain Acyl-CoA Dehydrogenase (VLCAD) Deficiency
Following the discovery of VLCAD in 1992, it became apparent that some patients thought to have a long-chain acyl-CoA dehydrogenase deficiency in fact had VLCAD deficiency ( ). Three phenotypes are associated with this deficiency: (1) a severe, often lethal, childhood form with early onset, cardiomyopathy and hypoglycaemia; (2) a milder childhood form with hypoglycaemia and dicarboxylic aciduria; and (3) a form resembling CPT II deficiency with rhabdomyolysis and myoglobinuria. Most patients suffer from the severe cardiomyopathic form. Muscle pathology associated with VLCAD deficiency is mild, with variation in fibre size and maintenance of a normal fibre type pattern ( Fig. 18.5 ). A mild increase in lipid staining may be seen.

Diagnosis is based on the finding of abnormal long-chain acylcarnitines in blood with a predominance of C 14:1 or immunohistochemical demonstration of VLCAD deficiency ( ) and mutation analysis of the gene ACADVL .
The elongation of long to very long fatty acids requires hydroxyacyl-CoA dehydratases, and mutations in one of these, encoded by HACD1 , are associated with congenital myopathy in humans and dogs (see Ch. 15 ) ( ).
Trifunctional Protein Deficiency
This protein is a hetero-octamer of four α-subunits with long-chain 2-enoyl-CoA hydratase and long-chain L-3-hydroxylacyl-CoA dehydrogenase (LCHAD) activities and four β-subunits with long-chain 3-ketoacyl-CoA thiolase activity. The α-subunits and β-subunits are encoded from the HADHA and HADHB genes, respectively. The majority of affected patients have a deficiency of LCHAD ( ). Age of onset ranges from neonatal to early childhood, and clinical manifestations include recurrent episodes of non-ketotic hypoglycaemia, sudden infant death, cardiomyopathy and myopathy. Muscle weakness is prominent in some children and associated with myoglobinuria and respiratory failure. Distinctive features of LCHAD deficiency are progressive pigmentary retinopathy and peripheral neuropathy. Mitochondrial proliferation, decreased respiratory chain activity and lipid accumulation may be found in skeletal muscle but are not consistent findings ( ) ( Fig. 18.6 ).

In a small group of patients all subunits of the mitochondrial trifunctional protein are deficient. Clinical features are similar to those described previously, and, although usually more severe, a few benign cases with recurrent exercise-induced rhabdomyolysis have been reported ( ). Increased levels of long-chain hydroxyacylcarnitines are present in blood during acute episodes.
Multiple Acyl-CoA Dehydrogenase (MAD) Deficiency (Glutaric Aciduria Type II)
This complex disorder, associated with electron transfer flavoprotein (ETF) and electron transfer flavoprotein coenzyme Q oxidoreductase (ETF:QO) deficiency, has different clinical presentations. Some patients present in infancy with renal cystic dysplasia and other congenital anomalies, and usually death occurs in the first few weeks ( ); other infants and children develop episodic hypoglycaemia, acidosis and hepatomegaly ( ); and a third group presents with muscle weakness ( ). The child described by Turnbull et al presented with severe muscle weakness at 6 months and responded well to treatment with a low-fat diet, riboflavin, carnitine and glycine ( ). Her brother had died at 3 months, probably with the same condition.
ETF is localized in the mitochondrial matrix as a heterodimer of α- and β-subunits, which are encoded by the ETFA and ETFB genes. ETF:QO is a monomer encoded by the ETFDH gene and is located in the inner mitochondrial membrane. It contains a 4Fe4S cluster as well as flavin adenine dinucleotide (FAD).
Morphological changes in muscle with lipid accumulation is frequently observed but is not a consistent finding ( Fig. 18.7 ). There are increased levels of acylcarnitines of several chain lengths in blood. Some cases respond to treatment with riboflavin. Mutations in ETFDH are the major cause of riboflavin-responsive MAD deficiency ( ).

Episodic Rhabdomyolysis Associated with TANGO2 Mutations
Biallelic mutations in the gene encoding the transport and Golgi organization 2, TANGO2 protein, are associated with episodes of metabolic crises frequently manifesting as rhabdomyolysis and with cardiac conduction defects and arrhythmias ( ). The onset is usually at infancy or early childhood, and the first symptoms can be an insidious onset of developmental delay and seizures or acute metabolic crisis with hypoglycaemia, hyperlactacidaemia and rhabdomyolysis. Cardiac manifestations include conduction defects, arrhythmias and in some cases dilated cardiomyopathy. Eventually, affected individuals develop brain atrophy and intellectual disability. The pathogenesis of TANGO2-associated disease is not established, since the function and localization of the protein have not been clarified. However, it has been suggested that β-oxidation is affected during metabolic crises ( ). Muscle pathology is characterized by unspecific changes related to rhabdomyolysis with necrosis and regeneration. Between the attacks there are no definite pathological changes.
Mitochondrial Myopathies
The mitochondrial myopathies are a heterogeneous and complex group of neuromuscular disorders in which abnormalities in mitochondrial metabolic function may or may not be associated with structural abnormalities in the mitochondria. Defects in enzymes encoded by nuclear genes or those of the mitochondrial genome can cause a myopathy. In addition, defects in some enzymes of the fatty acid pathway discussed previously can cause secondary structural abnormalities in mitochondria.
During recent years the field of mitochondrial myopathies has mushroomed on a number of fronts; the clinical, with recognition of an ever-increasing variety of presenting disorders, often with overlap of features between different syndromes; the genetic, with recognition of a maternal pattern of inheritance in some syndromes; the biochemical, with identification of specific biochemical abnormalities within the various complexes of the respiratory chain; and the molecular, with recognition of certain specific mutations in relation to the mitochondrial and nuclear genome. The situation has been further compounded by the fact that the same molecular abnormality may produce very divergent clinical features, and, in addition, there can also be a wide range in the severity of expression of a mutation within a single family. The mitochondrial myopathies belong to a group of multisystem diseases in which clinical and/or morphological evidence of myopathy is not always present.
Genetics
According to the endosymbiotic hypothesis, mitochondria originated from the fusion of eukaryotic cells with prokaryotic cells capable of oxidative phosphorylation about 1.5 billion years ago. Substrates are oxidized to H 2 O and CO 2 by oxidative phosphorylation, generating most of the ATP produced by the cell. The oxidative phosphorylation system, which is built up of five enzyme complexes, is embedded in the inner membrane of the mitochondria ( Fig. 18.8a ). The components of the oxidative phosphorylation system are encoded by two separate genetic systems, the nuclear and the mitochondrial genomes (see Fig. 18.8a, b ). The nuclear genome encodes most of the subunits of the enzyme complexes, assembly proteins and many of the factors necessary for mitochondrial DNA (mtDNA) replication, transcription and translation. The mitochondrial genome encodes 13 subunits of the oxidative phosphorylation (OXPHOS) system, as well as the ribosomal RNA (rRNA) and transfer RNA (tRNA) involved in the mitochondrial protein synthesis ( Fig. 18.9 ).


Mitochondrial disorders are caused by defective oxidative phosphorylation ( ). Diseases caused primarily by nuclear gene mutations show Mendelian inheritance, whereas diseases caused by primary mtDNA mutations are sporadic or show maternal inheritance as mitochondrial DNA is transmitted only from mother to child. One group of diseases with mendelian inheritance is caused by mutations in nuclear genes encoding proteins involved in mtDNA maintenance. In many of these cases the disease manifestations are caused by secondary mtDNA alterations such as multiple large-scale deletions and/or reduced copy number (mtDNA depletion).
Mitochondrial myopathy may also be acquired, as in the case of treatment with nucleoside reverse transcriptase inhibitors for HIV ( ) (see Ch. 23 ).
Morphological Aspects
Mitochondrial diseases are often characterized by mitochondrial myopathy and may be an important clue to correct diagnosis. Irrespective of the molecular background, mitochondrial proliferation is often present. The extreme variant of mitochondrial proliferation is referred to as ragged-red fibres (RRF) ( Fig. 18.10 ). The term ‘ragged-red’ was introduced by King Engel to describe the very disrupted fibres seen with the Gomori trichrome. These are characterized by marked proliferation of mitochondria, especially in the subsarcolemmal region, but also throughout the fibre. There is also lipid accumulation in the ragged-red fibres and capillary proliferation adjacent to them. Ragged-red fibres are associated with mitochondrial ultrastructural changes (see below). The mitochondrial proliferation is a segmental change, and the fibre may look completely normal at another level. In this chapter a mitochondrial myopathy is defined as a myopathy with abnormal mitochondrial proliferation and/or enzyme histochemical evidence of OXPHOS deficiency, without evidence of another primary muscle disease such as an inflammatory myopathy or a muscular dystrophy. It is important to know that in many primary mitochondrial diseases caused by either mitochondrial or nuclear gene mutations muscle morphology may be completely normal without any abnormal mitochondrial proliferation ( ).

Enzyme Histochemical Alterations
In mitochondrial myopathies there is deficiency of one or more of the five enzyme complexes that form the oxidative phosphorylation system. Enzyme histochemical methods are available for complex II (succinate dehydrogenase; SDH), complex IV (cytochrome c oxidase; COX) and complex V (ATP synthase). Although deficiency of mitochondrial ATP synthase can be demonstrated in some cases by enzyme histochemistry ( ), most diseases associated with mutations in genes coding for subunits of complex V do not show this enzyme histochemical deficiency. A mosaic pattern of COX-deficient and COX-normal fibres is indicative of a mtDNA defect ( Fig. 18.10c ). In order to readily recognize such muscle fibres, combined staining of both SDH and COX in the same section is very useful. The COX-deficient fibres stain blue in contrast to the normal fibres, which stain brown ( Fig. 18.10d ) ( ). When there is a generalized and evenly distributed histochemical deficiency of COX or SDH deficiency in the biopsy, the genetic cause is usually found in nuclear DNA.
In MELAS syndrome (mitochondrial encephalopathy, lactic acidosis and stroke-like episodes), strongly SDH-reactive blood vessels have been interpreted as a sign of mitochondrial vasculopathy ( ).
Immunohistochemical Alterations
Antibodies to proteins that build up the five enzyme complexes of the respiratory chain are available (see Ch. 6 ). Immunohistochemical studies on the respiratory chain enzyme complexes are useful when enzyme histochemistry is not possible in the case of formalin-fixed material or to get indications of complex I deficiency for which there is no enzyme histochemical method available ( ) ( Figs. 18.11 and 18.12 ).


Ultrastructural Alterations
In ragged-red fibres the number of mitochondria is increased and many of them also show various structural abnormalities ( Fig. 18.13 ). One such alteration is paracrystalline inclusions (see Fig. 18.13a ), which frequently show a parking lot appearance. In infants and young children, the paracrystalline inclusions are less frequent but, instead, the mitochondria display other structural changes such as enlargement and abnormal arrangements of the cristae (see Fig. 18.13b, c ; see also Ch. 5 ). In a systematic study on seven individuals with genetically defined mitochondrial myopathies, identified six major ultrastructural abnormalities, including paracrystalline inclusions, concentric cristae, linearized cristae with angular features, compartmentalization, nanotunnelling with tubular structures connecting mitochondria and hyperbranched mitochondria. In mitochondrial diseases without mitochondrial proliferation in muscle, the muscle mitochondria usually show normal structure. Therefore, the value of electron microscopy is usually limited to verification of mitochondrial pathology already observed by light microscopy ( ). In addition, ultrastructural alterations in mitochondria are frequently observed in many different myopathies that are not primarily OXPHOS disorders, and they may also be present in normal ageing.

Diagnostic Tools
As in all neuromuscular disorders, diagnosis of a mitochondrial disease is based on the clinical presentation and supported by additional investigations. In Tables 18.1 and 18.2 the more common mitochondrial syndromes are summarized. In many cases the diagnosis is not straightforward and a probable or definite mitochondrial disease will be diagnosed depending on the results of the investigations that are performed.
Syndrome | Major Clinical Features | Age at Presentation | OXPHOS Deficiency | Muscle Pathology | Genes |
---|---|---|---|---|---|
GRACILE | Fetal growth retardation,amino-aciduria, cholestasis, iron overload and lactic acidosis | Neonatal | Deficiency of complex III in some cases | Normal | BCS1L |
Leighsyndrome | Failure to thrive, hypotonia, psychomotor regression, brainstem dysfunction, ataxia, dystonia, optic atrophy | Infancy,sometimes later | Variable defects of OXPHOS Other defects such as PDH deficiency are common | Usually normal or COX deficiency without RRF | MT-ATP6 mt ND genes Nuclear ND genes SURF1 SDHA PDHA1 + many others |
Pearson syndrome | Failure to thrive, sideroblastic anaemia, pancytopenia, exocrine pancreas dysfunction, liver failure, renal tubulopathy | Infancy | Variable defects of OXPHOS | Normal but mitochondrial myopathy with COX-deficient RRF may be present | mtDNA deletions |
Alpers syndrome | Psychomotor deterioration, generalized seizures, infantile spasms, progressive microcephaly, spasticity, cortical blindness | Infancy | Combineddeficiency ofOXPHOS | Usually normal | NARS2 PARS2 FARS2 |
Alpers–Huttenlocher syndrome | Progressive encephalopathy, complex refractory seizures, epilepsia partialis continua, liver failure | Infancy and early childhood or young adulthood | Deficiency mainly of complex I or IV | Usually normal COX-deficient fibres may be present | POLG |
Myopathy of infancy | Muscle weakness, hypotonia, respiratory and feeding difficulties | Infancy | Combined deficiency of OXPHOS, mainly complex IV | Mitochondrial myopathy with or without COX-deficient RRF | TK2 RRM2B MT-TE |
IOSCA | Hypotonia, ataxia, ophthalmoplegia, sensorineural hearing loss, neuropathy, epileptic encephalopathy | Infancy or early childhood | Complex I and IV deficiency in neurons Normal in muscle | Normal | TWNK |
Syndrome | Major Clinical Features | Age at Presentation | OXPHOS Deficiency | Muscle Pathology | Genes |
---|---|---|---|---|---|
Isolated myopathy | Muscle weakness and wasting Exercise intolerance Myoglobinuria Cardiomyopathy in some cases | Childhood, adolescence or adulthood | Variable defects of OXPHOS | Mitochondrial myopathy with COX- deficient or COX-positive RRF SDH deficiency in some cases rhabdomyolysis and regeneration | MT-CYB mt COX genes mt tRNA genes ISCU FDX1L TK2 RRM2B |
MELAS | Short stature, migraine-like headache, generalized seizures, stroke-like episodes, dementia, ataxia, sensorineural hearing loss, muscle weakness, diabetes mellitus | Childhood, adolescenceor adulthood | Usually complex I deficiency, depending on mutation | Mitochondrial myopathy with COX-positive RRF | mt tRNA genes mt ND genes MT-CYB mt COX genes |
MERRF | Myoclonic and generalized seizures, ataxia, muscle weakness, sensorineural hearing loss | Childhood, adolescence or adulthood | Mainly combineddeficiency ofcomplexes I and IV | Mitochondrial myopathy with COX-deficient RRF | mt tRNA genes |
KSS | PEO, retinitis pigmentosa, cardiac conduction defects, short stature, sensorineural hearing loss, cerebellar ataxia, dementia, diabetes mellitus | Childhood, adolescence or adulthood | Variable defects of OXPHOS or normal | Mitochondrial myopathy with COX-deficient RRF | mtDNA deletions RRM2B |
MNGIE | Severe gastrointestinal dysmotility, cachexia, ptosis, ophthalmoparesis, peripheral neuropathy, leucoencephalopathy | Childhood, adolescence or adulthood | Complex IV deficiency or combined defects | Mitochondrial myopathy with COX-deficient RRF Neurogenic atrophy | TYMP RRM2B |
MLASA/ MSA | Muscle weakness, exercise intolerance, lactic acidosis anaemia | Childhood or adolescence | Combined defects | Mitochondrial myopathy with COX-deficient RRF | PUS1 YARS2 |
NARP | Muscle weakness, sensory neuropathy, ataxia, retinitis pigmentosa, dementia | Youngadulthood | Mainly normal | Normal | MT-ATP6 |
SANDO/MSCAE/MIRAS | Ataxia, sensory neuropathy Additional features may include PEO, migraine, severe epileptic seizures, myopathy, hearing loss | Childhood, adolescence or adulthood | Variable defects of OXPHOS or normal | Mitochondrial myopathy with COX-deficient RRF Sometimes normal | POLG |
ad/ar PEO | PEO, muscle weakness and exercise intolerance Additional features may include cardiomyopathy, parkinsonism, hypogonadism, encephalopathy | Adulthood | Variable defects of OXPHOS, which may even be normal | Mitochondrial myopathy with COX-deficient RRF and multiple mtDNAdeletions | POLG,POLG2 TWNK,OPA1 SLC25A4,TK2 DNA2,RRM2B MGME1,SPG7 RNASEH1 TK2 |
LHON | Rapid loss of central vision, optic atrophy | Usuallyadulthood | Complex Ideficiency | Normal | mt ND genes |
Investigation of mitochondria in muscle tissue has become an important tool in the diagnostic work up of patients in whom there is a suspicion of a mitochondrial encephalomyopathy. To enable the study of mitochondrial function and morphology, muscle biopsy is often performed. Common techniques to study the mitochondria in muscle tissue include enzyme histochemistry, electron microscopy, biochemical investigation of isolated fresh mitochondria by oximetry after the addition of various substrates and spectrophotometric analysis of respiratory chain enzymes and mtDNA analysis ( ). Additional techniques such as blue native gel electrophoresis to study protein subunits of the various complexes, Western blot analysis, Northern blot analysis, in situ hybridization, single-fibre mtDNA analysis and immunohistochemistry may be applied in certain situations. The combination of morphological and biochemical investigations is very helpful. For example, diseases due to severe complex I deficiency that can be revealed by biochemical investigation of muscle tissue frequently do not show any histochemical or structural alterations in muscle. On the other hand, mitochondrial diseases caused by large-scale mtDNA deletions often show clear morphological evidence of mitochondrial myopathy but frequently no biochemical defect as revealed by analysis of mitochondria in muscle homogenate. In cases of mitochondrial myopathy it is advisable to study mtDNA in muscle mitochondria, as mutations are sometimes only detected in muscle tissue, especially in cases of isolated myopathy.
Muscle Pathology in Relation to Underlying Genetic and Biochemical Defects
Muscle biopsy is often performed as part of an investigation of a suspected mitochondrial disease. It is therefore important to be aware of which mitochondrial diseases can be verified or excluded by morphological and enzyme histochemical analyses.
Primary Mitochondrial DNA Mutations
A flow chart describing diseases and morphological alterations associated with different mtDNA mutations is presented in Fig. 18.14 . In spite of the fact that mtDNA encodes only 13 polypeptides and nDNA encodes hundreds of proteins involved in the respiratory chain structure and function, the great majority of oxidative phosphorylation diseases with identified gene defect are due to mtDNA mutations in adults ( ). Mutations will only be transmitted from mother to child, since mtDNA is maternally inherited, but there are rare exceptions ( ). Although mtDNA with pathogenic mutations usually coexists with wild-type mtDNA, a phenomenon known as heteroplasmy, there are several examples of homoplasmic variants being pathogenic and heteroplasmic variants having no effect on protein function. Neutral polymorphisms are homoplasmic, with a few exceptions.

The proportion of mutant mtDNA copies is essential for the phenotypic expression of a mutation, and mtDNA mutations are therefore functionally recessive. When the mutant load exceeds a threshold level, the cell will be suffering from defective oxidative phosphorylation. The threshold level varies with different mutations but is usually high at the cell level (85–95%). Dominant negative effects of mtDNA mutations with low mutant load in affected tissues have been observed in rare cases ( ). Since mtDNA mutations are frequently restricted to one or a few tissues, analysis of blood samples may show no mutation. It is therefore important to analyze affected tissues. In diseases caused by mtDNA mutations, the mutation can usually be identified in muscle tissue. Cases of mitochondrial myopathy without other organ manifestations are frequently sporadic, and the mtDNA mutation can then only be found in muscle tissue, but not in cultured myoblasts.
Mutations of any of the protein-encoding genes typically cause deficiency of the corresponding complex, but combined deficiencies may be observed. Nevertheless, point mutations of tRNA genes, as well as large-scale deletions of mtDNA, cause impaired protein synthesis that affects all of the 13 polypeptides encoded by mtDNA. Therefore, combined deficiencies of the various complexes, except complex II of the respiratory chain, are common.
Single Large-Scale Deletions of mtDNA
Single large-scale rearrangements of mtDNA, deletions and/or duplications, are usually associated with progressive external ophthalmoplegia (PEO) ( ), frequently as part of the multisystem disorder Kearns–Sayre syndrome. Pearson’s syndrome, which is also associated with large-scale mtDNA deletions, includes early onset of sideroblastic anaemia and exocrine pancreas dysfunction ( ). Diseases caused by single large-scale rearrangements of mtDNA usually appear as sporadic cases. The risk for transmission of the mutation is approximately 4% ( ). There is usually mitochondrial myopathy and COX-deficient muscle fibres (see Fig. 18.10 ), but this may not be evident in Pearson’s syndrome.
Transfer RNA Point Mutations
Most pathogenic point mutations in mtDNA so far described affect tRNA genes. Maternally inherited mutations are common but de novo mutations also occur. Pathogenic mutations have been identified in most of the 22 tRNA genes. However, the MT-TL1 , MT-TI and MT-TK genes are more frequently affected than others. The most common tRNA mutation is m.3243A>G in MT-TL1 . This mutation was originally described in MELAS syndrome. Many other phenotypes have now been described with this mutation. The MELAS syndrome can also be caused by a large number of other mtDNA mutations, highlighting the overlap of clinical phenotypes and mtDNA molecular defects.
tRNA mutations are frequently expressed as multisystem disorders, but some mutations are more or less organ-specific with respect to clinical manifestations. Although tRNA mutations affect the translation of all mitochondrial encoded polypeptides, some mutations are typically associated with complex I deficiency, such as the MT-TL1 mutations , whereas others are associated with deficiency of complex III ( MT-TY ) ( ) or complex IV ( MT-TW ) ( ). Diseases caused by tRNA mutations are usually, but not always, associated with mitochondrial myopathy (see Fig. 18.11 ). Since there is a predominant deficiency of complex I in many cases associated with MT-TL1 mutations, such cases show mitochondrial myopathy with COX-positive ragged-red fibres ( Fig. 18.15 ).
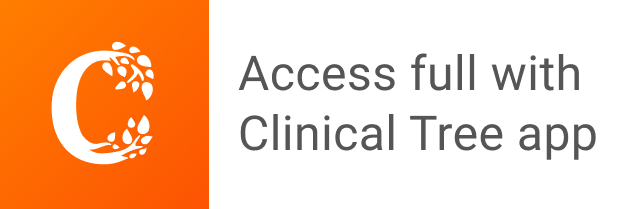