Pearls
- •
Modern respiratory care requires a major institutional commitment in resource allocation for state-of the-art management of the pediatric patient requiring mechanical ventilation.
- •
A comprehensive and unified approach in which the roles and responsibilities of each of the team members (physicians, respiratory therapists, bedside nurses, and the family) are clearly defined and respected is required.
- •
Every team member must be aware of the current technologic advances in the design and implementation of mechanical ventilation as well as the unique physiology of pediatric patients.
This chapter reviews the applied physiology relevant to mechanical ventilation and design and function of ventilators, including a modern classification of the modes; guidelines for the use of mechanical ventilation based on pathophysiology, philosophy, and practice of weaning; respiratory care adjuncts to mechanical ventilation; high-frequency ventilation; and the adverse effects of mechanical ventilation. The topic of noninvasive mechanical ventilation, including negative-pressure ventilation, is covered in Chapter 55 .
Applied respiratory physiology
This section reviews principles of respiratory physiology necessary to understand and optimize mechanical ventilation. A more in-depth description of respiratory physiology is available in Chapters 42 , 45 , and 46 .
Lung volumes and capacities
Breathing fulfills the physiologic necessity of gas exchange. Oxygen is delivered to the blood with each inspiration, and carbon dioxide is removed with each exhalation. Lung volumes increase during inspiration and fall during expiration ( Fig. 54.1 ). Tidal volume (V t ) is the volume of gas that moves in and out of the lungs with each breath and is normally 5 to 8 mL/kg for a spontaneous breath, regardless of age. Total lung capacity (TLC) is the volume of gas present in the lung with maximal inflation (60–80 mL/kg). Vital capacity is the volume of gas that can be maximally expired from TLC (40–50 mL/kg). Residual volume is the volume of gas present in the lung at the end of a maximal expiratory effort and represents anatomic dead space. Functional residual capacity (FRC) is the volume of gas that is present in the lung at the end of a normal expiration. FRC results from the balance between forces that favor alveolar collapse and those that maintain alveolar inflation, with normal FRC being approximately 30 mL/kg. Closing volume refers to the volume of gas present in the lung at which small conducting airways begin to collapse. When FRC exceeds closing volume, the small airways and alveoli remain open. On the other hand, when closing volume exceeds FRC, the small airways tend to collapse, followed by the alveoli, owing to the continued absorption of gases into the bloodstream. Because of the highly compliant chest wall of infants and children younger than 6 years, the closing volume can exceed FRC. This is one reason for the propensity for atelectasis in infants and young children. With development, FRC exceeds closing volume in children older than 6 years.

Lung inflation and deflation
Thoracic structures impede lung inflation; a certain amount of force is required to overcome this impedance. Elasticity of the lung and chest wall is typically the primary factor that resists lung inflation, and respiratory system compliance dictates how much pressure is required to deliver a certain V t to the lungs. Total respiratory system compliance is a sum of the compliance of both the lungs and chest wall. Disease processes that result in an abnormal lung or chest wall compliance fall into the category of restrictive lung disease as listed in Table 54.1 .
Restrictive Lung Disease | ||
---|---|---|
Decreased Lung Compliance | Reduced Chest Wall Compliance | Obstructive Lung Disease (Increased Resistance) |
|
|
|
Total respiratory system resistance is the second factor that impedes inflation. Resistance is defined as the pressure required to produce flow into the lungs. It can be partitioned into airway resistance and frictional resistance to deformation of the lungs, chest wall, and abdominal contents (also known as nonelastic frictional resistance). In the infant, airway resistance is equally distributed between the upper and lower airways. However, with increasing age, most of the airway resistance resides in the upper airways. Airway resistance is primarily a function of the caliber of the airways but is also dependent on the viscosity and density of the gas and whether the gas flow is in a laminar or turbulent state. In obstructive lung disease, airway resistance can be dramatically increased in the setting of bronchospasm, upper airway edema or fixed obstruction, and airway malacia (see Table 54.1 ). In certain pathologic conditions—such as pulmonary edema, interstitial lung disease, and pulmonary fibrosis—frictional resistance may also be increased.
Normal expiration is passive and dependent on the elastic recoil of the lung, which is attributable to alveolar surface tension and tissue elasticity. Surface tension is greatest at high lung volumes and lowest at FRC. Elastic recoil of the lung provides most of the force required to expel the gas from the lungs, but expiration may be aided by respiratory muscles during forced exhalation. Airway resistance will impede expiration in that higher resistance will slow the flow of gas out of the lungs until closing volume is reached and airflow will cease.
Time constant
Understanding the concept of time constants is necessary to optimize ventilator settings, particularly ventilation rate. With a constant inflation pressure, it takes a finite amount of time to inhale or exhale a given volume of gas. The rate of inflation and deflation of the lung is approximately mono-exponential and is directly proportional to compliance and resistance. Inspiratory and expiratory time constants will be different as the inspiratory and expiratory resistances differ. Time constant is calculated as the product of compliance and resistance. Each time constant represents the amount of time required for 63% of change in lung volume. As such, it takes one, three, and five expiratory time constants to reach a 63%, 95%, and 99% exhalation, respectively ( Fig. 54.2 ).

Work of breathing
During normal breathing, work of breathing is performed entirely by inspiratory muscles. Nearly half of the work of breathing during normal inspiration is dedicated to overcoming frictional resistance. The remaining inspiratory work is stored as potential energy that is used to perform the expiratory work. Increased airway resistance and decreased chest and lung compliances require a greater pressure to inflate the lung to the same lung volume, imposing a greater workload on the respiratory muscles and increasing the energy cost of breathing. When the oxygen supply-demand balance to the respiratory muscles is perturbed, respiratory failure may ensue secondary to muscle fatigue.
Determinants of gas exchange
The determinants of systemic arterial oxygenation are multifactorial and include inspired oxygen concentration and tension, lung volume, cardiac output, and ventilation-perfusion (V/Q) matching. Alveolar collapse leads to inadequate oxygenation owing to increased physiologic dead space and intrapulmonary shunting resulting from V/Q mismatch. During expiration, the presence of alveolar surfactant helps prevent alveolar collapse. Surfactant deficiency, loss, or alteration promotes alveolar collapse and increases the critical opening pressure of the alveoli. Parenchymal lung disease is also characterized by an increased critical closing pressure and alveolar collapse. Alveolar diseases, including pneumonia and acute respiratory distress syndrome (ARDS), affect oxygenation owing to both alveolar collapse and increased resistance to gas diffusion across the alveolar basement membrane. Chapters 48 , 51 , and 52 contain further descriptions of these pathologic states.
The determinants of systemic partial pressure of arterial carbon dioxide (Pa co 2 ) are a balance between CO 2 production (metabolic rate) and CO 2 elimination (minute ventilation, the product of V t and respiratory rate). Decreased CO 2 elimination usually results from decreased central drive, airway obstruction, parenchymal disease, and muscle weakness. Sufficient pulmonary blood flow is also necessary for CO 2 elimination; states of reduced pulmonary blood flow (such as pulmonary hypertensive crisis or cardiac arrest) will also result in elevated Pa co 2 . Increased metabolic production of CO 2 usually is caused by hypermetabolic states or excessive caloric intake, especially high-carbohydrate alimentation.
Indications for mechanical ventilation
Respiratory failure
The primary indication for institution of assisted ventilation is respiratory failure, which is generally defined as the presence of inadequate oxygenation, inadequate ventilation, or both. Apnea or respiratory arrest is an extreme form of respiratory failure; prolonged apnea is an indication for immediate mechanical ventilation. In critically ill children, establishing mechanical ventilation before respiratory failure develops is preferable while the patient maintains some physiologic reserve and is less likely to suffer clinical deterioration—and, potentially, cardiac arrest—during the intubation process.
Inadequate oxygenation , objectively, is defined as a partial pressure of arterial oxygen (Pa o 2 ) less than 60 torr, an arterial hemoglobin oxygen saturation less than 90% in room air, or a Pa o 2 :F io 2 (fraction of inspired oxygen) ratio less than 300. Other indices include an alveolar-to-arterial oxygen gradient of greater than 300 torr with F io 2 of 1.0. However, adequacy of oxygenation should be assessed in terms of the patient’s global disease state and oxygen delivery rather than relying solely on an objective cutoff. Inadequate oxygenation owing to intrapulmonary shunting can typically be overcome with the addition of increased inspired oxygen concentration provided that the magnitude of the shunt is less than 15%. Intrapulmonary shunt can be decreased by reexpanding collapsed alveoli or decreasing the fraction of pulmonary blood flow distributed to the collapsed/consolidated alveolar segments.
Inadequate ventilation is objectively defined as a Pa co 2 greater than 45 torr with an arterial pH less than 7.35 in the absence of chronic hypercapnia. Acute-on-chronic ventilatory failure is objectively defined as a change in Pa co 2 of at least 20 torr with a corresponding decrease in arterial pH. Impending respiratory failure—characterized by rapidly rising Pa co 2 , progressive respiratory distress, progressively poor oxygenation, Pa co 2 out of proportion to the respiratory effort, or fatigue of respiratory muscles is a relative indication for mechanical ventilation. Chronic respiratory failure is defined as the need for mechanical ventilation for more than 28 days.
Other indications for mechanical ventilation
Just as certain disease processes (e.g., shock) may have increased oxygen demands and benefit from early mechanical ventilation, there may be relative indications for mechanical ventilation independent of impending respiratory failure. Children may require mechanical ventilation for airway protection, cardiac disease or failure, neurologic impairment or sedation needs, respiratory muscle weakness, and even to reduce caloric needs to optimize nutritional status and allow growth. In this manner, clinicians must use their clinical judgment to augment objective definitions of respiratory failure. Chapter 44 provides a deeper discussion of the many causes of breathing failure.
Design and functional characteristics of ventilators
A ventilator is simply a machine that performs external work to support a patient’s breathing. Energy applied to the device is altered, transmitted, and directed in a predetermined manner to either augment a patient’s breathing efforts or replace the patient’s work of breathing completely. Ventilators move gas into the lungs by increasing transpulmonary pressure (P TP ). Positive pressure ventilators create P TP by raising the airway pressure (P aw ) above the intrapleural pressure (P pl ), whereas negative-pressure ventilators create P TP by decreasing P pl below P aw . During mechanical ventilation, P TP may be generated by the ventilator, respiratory muscles during a spontaneous breath, or a combination of both.
All ventilators include input power, a drive system, control system, cycling mechanism, and a system to provide positive end-expiratory pressure (PEEP). Common accessories include a heated humidifier and oxygen blender. The input power to operate the ventilator is usually electric or pneumatic. The drive system provides the pressure gradient between the ventilator and lungs required to generate a gas flow, typically using compressed gases at high pressures from wall outlets, cylinders, or a small compressor designed to be used with individual ventilators. In this scheme, the ventilator acts only to modulate the flow of gas and will not function if the external pressurized source of gas fails. A ventilator with an internal compressor does not need an external source of gas to inflate the lung. A detailed review of the physical characteristics and functional design of ventilators is beyond the scope of this chapter; the reader is referred to several excellent reviews on this subject.
Pressure, volume, flow, and timing are the primary variables that the provider may control with any mechanical breath; these variables will be explored in more detail later. It is important to note that these variables are not independent of each other and that each is also dependent on respiratory system compliance and resistance.
Phases of a breath
A breath is defined as one cycle of positive flow (inspiration) and negative flow (expiration). The key components of a mechanical breath are the trigger, flow pattern, limit, cycle, and inspiratory time ( Fig. 54.3 ). Trigger is the signal to initiate the mechanical breath, flow pattern describes how the air flows into the patient during inspiration, limit describes the parameter used to terminate lung inflation, and cycle is the parameter that allows lungs to empty. While each ventilator may have unique proprietary names for different ventilator modes, defining the trigger, flow pattern, limit, and cycle will allow the bedside provider to understand how each breath is delivered to the patient.

Inspiratory time is defined as the period from the start of positive flow (into the patient) to the start of negative flow (out of the patient). Expiratory time is defined as the period from the start of negative flow to the start of positive flow. Total cycle time is the sum of inspiratory and expiratory times and is equal to the inverse of breathing frequency. The inspiratory-expiratory (I : E) ratio is defined as the ratio of inspiratory time to expiratory time. Percent inspiratory time (also called the duty cycle ) is defined as the ratio of inspiratory time to total cycle time. Delivered V t is the integral of flow with respect to time.
Initiating breaths
Trigger is the signal that starts inspiration and can be initiated by either the ventilator or the patient (see Fig. 54.3 ). Time, pressure, flow, minimum minute ventilation, apnea interval, and electrical signals (e.g., diaphragmatic activity) can all be used as inspiratory triggers. When inspiratory flow starts with a ventilator-generated signal, it is referred to as a ventilator- or time-triggered breath and is independent of a patient-initiated signal. Similarly, when inspiration starts on a patient-generated signal (e.g., initiated by a change in flow or pressure), it is referred to as patient triggered and is independent of a ventilator-generated signal. Flow or electrical triggers are typically the most sensitive and will allow for improved synchrony, particularly in infants or patients with weak diaphragmatic effort. ,
Patterns of gas flow
The pattern of gas flow delivered is determined by an adjustable resistance valve on the driving pressure within the ventilator. Airway pressure and lung volume will increase until inspiratory flow is terminated. Four distinct inspiratory flow patterns are recognized: (1) constant flow, (2) decelerating flow, (3) accelerating flow, and (4) sinusoidal or sine-wave flow ( Fig. 54.4 ). Constant flow will increase pressure and volume linearly, while decelerating flow will result in rapid pressure and volume rise at the beginning of a breath, which then slows toward the end of inspiration (see Fig. 54.4 ). Decelerating flow is most commonly used, as it mimics natural breathing and is likely to meet patient flow demands. However, different flow patterns may be beneficial for specific disease processes. A sine-wave or sinusoidal inspiratory flow is created when the drive mechanism is a rotary wheel–driven piston. Accelerating flow and sine-wave flow patterns are rarely used by ventilators in current practice. During exhalation, expiratory flow curves depend on the elastic recoil of the lung in combination with airway resistance as well as the expiratory resistance in the system, including the PEEP valve.

Limit
During assisted breathing, the ventilator will continue to deliver flow into the patient until a predetermined limit is reached. The most commonly used limits are pressure and volume. In volume-limited ventilation, the ventilator will deliver a set volume of gas and the peak inspiratory pressure required to deliver that volume will be variable. In pressure-limited ventilation, the ventilator will provide flow until a set peak inspiratory pressure is reached, with variable tidal volumes. A flow-limited breath will terminate flow into the patient at a predetermined peak inspiratory flow. Just because the limit is reached and flow into the patient ceases, gas may not necessarily be allowed to leave the patient, as the lungs are held in an inflated state until the beginning of expiration (cycling).
Cycling of breaths
Cycling refers to the termination of inspiration and start of expiration (see Fig. 54.3 ). Inspiratory time, pressure, volume, flow, and electrical signals are all used as cycling signals. Ventilator cycling refers to ending inspiration based on signals from the ventilator (typically, a provider-set inspiratory time) independent of signals based on patient factors. Patient cycling occurs when a patient’s respiratory mechanics reaches a threshold value. For example, in flow cycling, which is a form of patient cycling, the ventilator transitions into the expiratory phase once inspiratory flow decays to a threshold value dependent on the patient’s respiratory mechanics (commonly, 10%–25% of the peak expiratory flow for the breath).
Continuous positive airway pressure and positive end-expiratory pressure
In lung diseases characterized by nonuniform or heterogeneous parenchymal involvement, FRC may be reduced below closing volume, resulting in atelectasis. These closed alveoli will have higher alveolar surface tension and therefore reduced compliance compared with open alveoli. This discrepancy in surface tension will have two effects: (1) V t will preferentially be delivered to open alveoli, potentially causing hyperinflation of normal lung segments and associated volutrauma ( Fig. 54.5 ); and (2) recruitment of these collapsed alveoli requires higher airway pressures than those needed to sustain inflation once the alveoli are open, and the reopening of these alveoli may cause shear injury to alveolar cells, a process known as atelectrauma.

Continuous positive airway pressure (CPAP) refers to the maintenance of positive airway pressure throughout the respiratory cycle with no positive-pressure breaths being delivered to the patient. Positive end-expiratory pressure (PEEP) refers to the maintenance of positive airway pressure above atmospheric pressure between breaths. The goals of CPAP and PEEP are to (1) increase end-expiratory lung volume above closing volume to prevent alveolar collapse, (2) maintain stability of alveolar segments, (3) improve oxygenation through increasing mean airway pressure and improving V/Q mismatch, and (4) reduce the work of breathing.
Ventilator modes and phase variables
All modern ventilators provide synchronized intermittent mandatory ventilation (SIMV) in that, when possible, the ventilator uses a patient trigger to match the patient’s desire for a breath to an actual delivered breath. This synchronization improves patient comfort, reduces air hunger, and allows for optimal lung mechanics.
In standard ventilator taxonomy, a control mode means that the limit and inspiratory time are predetermined, while a support mode implies that only the limit may have been preselected. The set limit will then determine the taxonomy, that is, volume control describes controlled breaths with a volume limit and pressure support describes supported breaths with a pressure limit. Control breaths may also be referred to as mandatory breaths, and support breaths may also be referred to as spontaneous breaths, in which the patient determines the timing of breath initiation and cycling. A support breath may be assisted (i.e., patient triggered with an additional positive pressure to a set limit) or unassisted (i.e., without any additional ventilator assistance, as might be seen in breathing during CPAP). It is important to understand that these terms—for example, volume control (VC-SIMV), pressure control (PC-SIMV), pressure support (PSV)—are standardized based on general taxonomy and do not refer to a specific manufacturer’s branded mode. It should be noted that a mixed mode is commonly used for ventilating patients (e.g., volume control/pressure support).
In a pure control mode (e.g., assist control), each breath will be a control breath with a predetermined inspiratory time. In a mixed mode, the patient will receive a set number of control breaths (determined by the set ventilator rate) with additional patient-triggered breaths above that rate delivered as supported breaths. In either of these modes, if the patient goes too long without triggering a breath, the ventilator will deliver a time-triggered control breath. The delay until that time-triggered breath occurs is dependent on the set ventilator rate as well as the number of breaths delivered over a set period of time, as determined by the ventilator’s programmed algorithm. If the patient does not trigger any breaths, the ventilator will deliver control breaths at the set ventilator rate. In a support mode, only spontaneous, patient-triggered support breaths will receive mechanical support. However, almost all ventilators are equipped with an apnea setting that will transition to a set rate if the patient is breathing below the alert backup rate.
Selection of parameters for mandatory breaths
Table 54.2 summarizes approximate initial settings for traditional ventilator modes. The first parameter typically targeted is the V t . The actual V t delivered to the patient is known as the effective tidal volume (V t eff ), which can be approximated by the following formula:
Tidal volume (V t eff ) | 5–8 mL/kg ideal body weight 3–6 mL/kg ideal body weight in states of reduced lung compliance |
Plateau pressure (P plat ) | ≤28 cm H 2 O ≤32 cm H 2 O in children with reduced chest wall compliance |
PEEP | 5–8 cm H 2 O |
Rate (IMV) a | Infants: 25–30 breaths/min Children 1–6 years: 20–25 breaths/min Children >6 years: 12–20 breaths/min |
Inspiratory time | Infant: 0.4–0.7 s Adolescent: 0.7–1.2 s Allow I:E ratio of at least 1:2 |
where V t del is V t delivered by the ventilator and V t circuit is the volume of gas that is distributed to the ventilator circuit.
A desirable V t eff for most mechanically ventilated children is somewhere between 5 and 8 mL/kg, as measured at the endotracheal tube. , Because dead space in the ventilator circuit plays a proportionally larger factor in smaller children, a discrepancy will exist between the targeted ventilator V t del and actual V t eff . V t may be directly set in a volume-limited mode or Δ-pressure (difference between peak inspiratory pressure and PEEP) may be titrated to achieve that volume in a pressure-limited mode. To avoid barotrauma, the end-inspiratory alveolar pressure (i.e., plateau pressure or pause pressure) should be targeted no higher than 28 cm H 2 O (or 32 cm H 2 O in patients with reduced chest wall compliance). , The plateau pressure can be measured using an inspiratory hold maneuver. With an inspiratory hold, both inspiratory and expiratory valves are closed, all flow is stopped, airway resistance is removed from the pressure equation, and the proximal airway pressure equilibrates with the alveolar pressure. Large V t and high peak pressures can each contribute to ventilator-associated lung injury through volutrauma and barotrauma, respectively. To avoid this injury, smaller V t (3–6 mL/kg) may be targeted in patients with reduced lung compliance. ,
PEEP is generally the next parameter selected. This level will depend on the clinical circumstances. The optimal PEEP is the level at which there is an acceptable balance between the desired clinical goals and undesired adverse effects. Clinicians should target PEEP to optimal lung expansion and to maintain adequate Pa o 2 or Sa o 2 (arterial oxygen saturation) to meet clinical goals with a “nontoxic” inspired oxygen concentration. , Arbitrary limits cannot be placed on the level of PEEP or mean airway pressure required to maintain adequate gas exchange.
In conjunction with PEEP, F io 2 is adjusted to maintain an adequate Sa o 2 and Pa o 2 . High concentrations of oxygen can contribute to lung injury from development of free radicals and should be avoided. The exact threshold of inspired oxygen that increases the risk of lung injury is not clear, but an F io 2 less than 0.5 is generally considered safe. In patients with parenchymal lung disease with significant intrapulmonary shunting, the major determinant of oxygenation is lung volume, which is a function of the mean airway pressure. With a shunt fraction of more than 15%, oxygenation may not be substantially improved by higher concentrations of oxygen; lung recruitment with PEEP will be more impactful.
Ventilator rate is selected based on the age and ventilatory requirements of the patient; subsequently, it may be adjusted according to the Pa co 2 . The inspiratory time is selected to provide an inspiratory-to-expiratory time (I:E) ratio of at least 1 : 2 in most patients. Inspiratory time can be set as either a percentage of the total respiratory cycle or as a fixed time in seconds depending on the ventilator. Inspiratory time must be selected to allow sufficient time for all lung segments to be inflated. In heterogeneous lung disease with varying regional time constants, a short inspiratory time may not be sufficient to inflate all lung segments and may contribute to underventilation and underinflation. Similarly, sufficient expiratory time must be provided for all lung segments to empty. If inspiration starts before the lung has completely emptied, gas trapping and inadvertent intrinsic PEEP will result. In infants with bronchiolitis and children with asthma, the expiratory time commonly must be lengthened to avoid gas trapping ( Fig. 54.6 ). This is accomplished most effectively by decreasing the respiratory rate rather than by excessively shortening the inspiratory time.

Dual-control modes
Dual-control modes of ventilation adjust the targeted limit on a breath-to-breath basis based on feedback from the patient’s respiratory mechanics. In pressure-regulated volume control (PRVC), adaptive pressure ventilation (APV), and variable pressure control, delivered V t is used as a feedback control for continuously adjusting the pressure limit. In these control ventilation modes, the provider selects a target V t , and the ventilator calculates the necessary pressure based on system compliance on a breath-to-breath basis. The ventilator will alarm if the V t and maximum pressure limit settings are incompatible. These modes may be of particular benefit in patients with rapidly changing compliance. Volume support and variable pressure support are terms used to describe this same strategy with a support mode of ventilation.
Modes such as average volume assured pressure support (AVAPS) and pressure augmentation allow the ventilator to switch from a pressure-controlled breath to a volume-controlled breath within the breath. These modes can be considered a safety net that always supplies a minimum V t . Once the breath is triggered, the ventilator attempts to reach the pressure-support setting as quickly as possible. As this pressure level is reached, the ventilator’s microprocessor determines the volume that has been delivered from the machine, compares this measurement with the desired V t , and determines whether the minimum desired V t will be reached. If the ventilator determines that the ultimate delivered V t will be equivalent to the set V t , the breath is delivered as a pressure-support breath. If the ventilator determines that the ultimate delivered V t will be less than the set V t , the breath changes from a pressure-limited to a volume-limited breath.
Automatic tube compensation
Automatic tube compensation (ATC) is a technique for overcoming the imposed work of breathing caused by the increased resistance of artificial airways. This is accomplished by using the known resistive characteristics of the artificial airways. ATC is essentially pressure support in which the ventilator adjusts the delivered pressure to compensate for the imposed work of breathing by the artificial airways and the flow demand of the patient. To select the level of ATC required, the operator inputs the type of tube, endotracheal or tracheostomy, and percentage of compensation desired (10%–100%).
Nontraditional modes of ventilation
Airway pressure release ventilation
Airway pressure release ventilation (APRV) is a mode of mechanical ventilation used to improve oxygenation by maintaining an open lung with high mean airway pressures while allowing spontaneous breathing. Additional ventilatory assistance is provided with intermittent release breaths, allowing exhalation below the target mean airway pressure for brief moments. APRV is essentially pressure-control ventilation using an inverse I:E ratio, with spontaneous breaths allowed during the mandatory breaths ( Fig. 54.7 ).

The mandatory breaths applied by APRV are time-triggered, pressure-targeted, time-cycled breaths. Minute ventilation occurs with both spontaneous breaths and the periodic inflation and deflation that occur between the two levels of airway pressure. Four major variables determine an APRV breath: P high , P low , T high , and T low . P high is defined as the pressure of the triggered mandatory breath, and T high is the duration that P high is maintained. P low is the release pressure, and T low is the time of the pressure release. P high is the primary determinant of mean airway pressure and oxygenation; T high is inversely proportional to the number of release breaths and affects both carbon dioxide removal and, to a lesser extent, mean airway pressure. P low is typically set 0 to 5 cm H 2 O to allow lung recoil during a release breath, yet T low is set short enough that alveolar collapse does not occur before lung reinflation. Currently, some modern ventilators have incorporated features that allow spontaneous breathing through a demand-flow system during both the inspiratory and expiratory phases of a pressure-controlled, time-cycled breath. These may be called by different names, such as biphasic positive airway pressure and intermittent mandatory pressure-release ventilation but are still classified under the acronym APRV. Some ventilators also allow pressure support to be provided for the spontaneous breaths during these modes.
APRV has not been well studied in children. Most of the articles published on this mode are case reports or small case series, mainly as a rescue therapy for refractory hypoxemia. Most of the studies have shown improvement in oxygenation, likely related to the higher mean airway pressure with APRV. ,
Proportional assist ventilation
Proportional assist ventilation (PAV) is a mechanical ventilation mode that relies on the instantaneous elasticity and flow resistance of the respiratory system. When PAV is initiated, the ventilator provides “test breaths” (controlled breaths with fixed flow and volume), which allows estimation of the respiratory system mechanics, minute ventilation, and work of breathing (resistive and elastic ventilator muscle loads). These are repeated at regular intervals to recompute the load estimations. These estimations provide inputs for the PAV algorithm. The clinician then sets the level of PAV (usually a percentage of the total work) to be provided breath by breath by the ventilator.
Unlike conventional breaths—in which volume, flow, or pressure is predetermined—in PAV the ventilator applies the pressure in relation to the patient effort after the breath has started. As the patient makes an inspiratory effort, the ventilator monitors the instantaneous rate and volume of gas flow, and then amplifies the patient’s effort during the breath by applying additional pressure. PAV can be conceptualized as simply continuously adjusting pressure support ventilation (PSV), in which the level of pressure support is attuned as a multiple of the sum of the volume and flow signals. The few clinical studies in infants and children demonstrate that PAV can maintain gas exchange with lower airway and transpulmonary pressure and improve thoracoabdominal synchrony but no differences in clinical outcomes.
Neurally adjusted ventilatory assist
Neurally adjusted ventilatory assist (NAVA) is a mode of PSV in which changes in electromyographic activity of the diaphragm provide the trigger and determine the level of support for each breath. In NAVA, the ventilator assists each spontaneous breath with pressure that is proportional to the integral of the electrical activity of the diaphragm (EAdi). NAVA uses a specifically designed catheter to measure the transesophageal recording of EAdi. For NAVA to be effective, it is critical that the esophageal catheter measuring EAdi be positioned correctly. The EAdi signal is used to both trigger and cycle-off the breath; the magnitude of ventilator support is determined by a mathematic function that represents diaphragmatic electrical activity times a gain factor, as shown by the equation
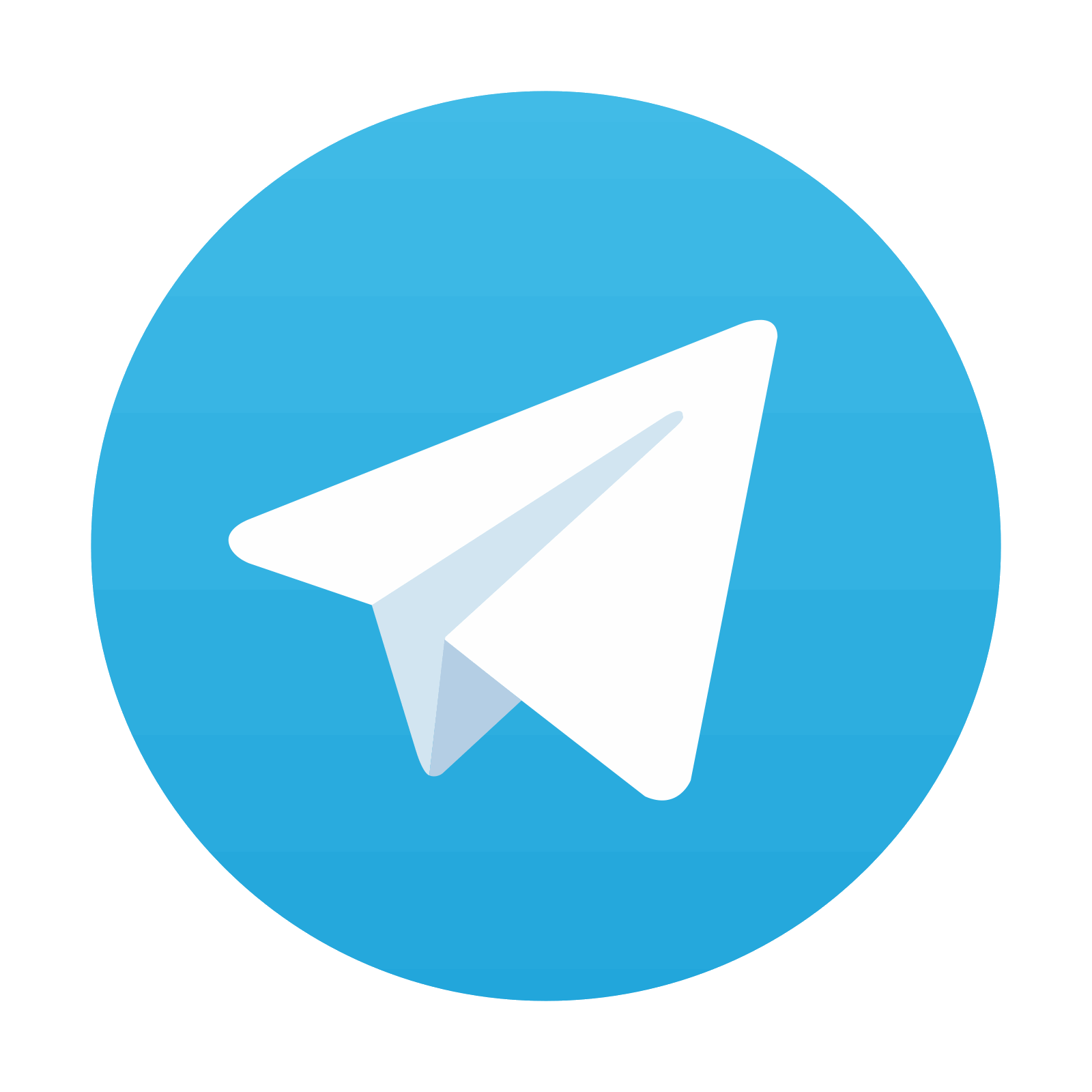
Stay updated, free articles. Join our Telegram channel
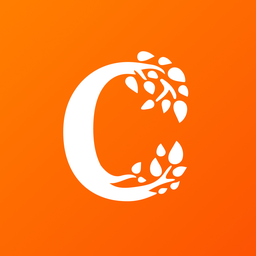
Full access? Get Clinical Tree
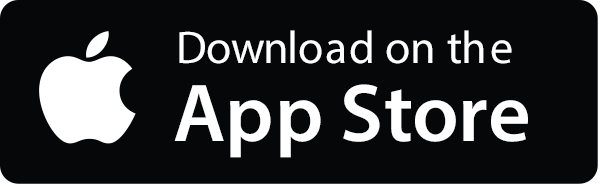
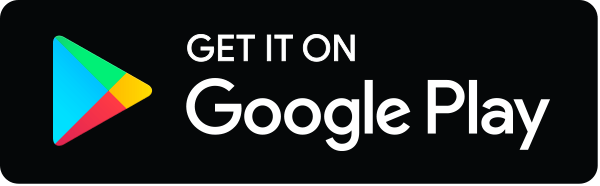
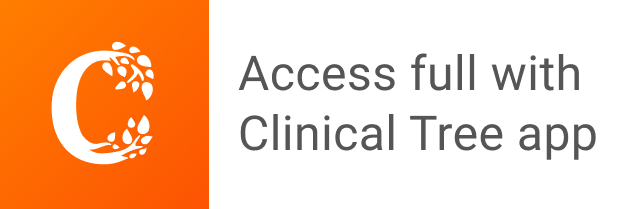