MRI Basics
Magnetic resonance imaging (MRI) provides a noninvasive means by which to achieve excellent visualisation of soft tissues with additional sensitivity to pathological bone marrow changes within the knee. Exploitation of inherent tissue relaxation characteristics allows for image sets with different contrasts to be generated. Relaxation in this context refers to the process of hydrogen nuclei (spins) returning to their equilibrium status after excitation with a radiofrequency (RF) pulse. After the application of an RF pulse, there is a restoration of initial magnetisation along the direction of the main magnetic field (B 0 ). On termination of the RF pulse, T1 relaxation time is the time constant that characterises the rate at which the initial magnetisation along the longitudinal (z) axis is recovered, whereas T2 relaxation time is the time constant for the decay of magnetisation in the transverse (x-y) plane. T1 and T2 characteristics are largely determined by a tissue’s inherent structural characteristics, which affect the ability of hydrogen protons (spins) to interact with each other (e.g., spin-spin or T2 relaxation) and the exchange of their excited kinetic energy to macromolecules in the surrounding environment (e.g., spin-lattice or T1 relaxation). ,
Conventional MRI Techniques
Modification of acquisition parameters such as the interval between RF pulses (repetition time; TR), or the interval from RF pulse to the sampled signal (echo time; TE) produces alterations in soft tissue contrast (weighting) within generated images. Although the ideal image weighting is dependent on the tissue being imaged, clinical musculoskeletal MR imaging protocols often use a combination of T1, T2 and proton density (PD) weighted images along with fat-suppressed, fluid-sensitive images that allow for detection of mobile water. PD weighted fast spin-echo (FSE) sequences paired with a fat-suppressed, fluid-sensitive sequence constitute the clinical ‘workhorses’ for orthopaedic assessment of the knee because they provide superior contrast among different tissue types and allow for detection of fluid and oedema ( Table 3.1 ).
Series | TR (ms) | TE (effective ms) | BW (kHz) | ETL | FOV (cm) | Slice Thickness (mm) With No Gap | Matrix | NEX |
---|---|---|---|---|---|---|---|---|
Sagittal FSE (cartilage sensitive) | 4000–4500 | 34 | 32 | 8–12 | 16 | 3.5 | 512 × 384 | 2 |
Coronal FSE (cartilage sensitive) | 4000–4500 | 34 | 32 | 8–12 | 11–13 | 3.0 | 512 × 256–320 | 2 |
Axial FSE (cartilage sensitive) | 4500 | 34–40 | 32 | 10 | 14 | 3.5 | 512 × 256–382 | 2 |
Sagittal FSE (fat suppressed) | 3500–4000 | 40 | 20.8 | 8–12 | 16 | 3.5–4 | 256 × 224 | 2 |
Cartilage
Articular cartilage is composed of primarily water (80%), with proteoglycans (PG) and collagen constituting just 20% of its total wet weight. The mechanical behaviour of cartilage can be attributed to interactions among these primary matrix components: water (retained by PG) provides resistance to compressive loads, whereas collagen fibrils within the cartilage matrix resist shear forces and provide tensile strength. Articular cartilage can be divided into four distinct zones: (1) the superficial zone, (2) the transitional zone, (3) the radial zone and (4) the calcified zone. Zonal variations in collagen architecture and PG concentration throughout the depth of knee cartilage produce attendant variations in signal intensities ( Fig. 3.1 ). Compared with all other (deeper) zones, the superficial zone has the highest collagen to PG ratio and water content and appears as a thin, relatively hypointense layer. Generally, tissues with a higher-ordered ultrastructure (such as cortical bone or menisci composed primarily of type I collagen) yield shorter T2 relaxation and therefore lower signal on most traditional MRI sequences. The transitional zone has higher PG content and lower water and collagen content compared with the superficial zone but more water and PG content compared with the radial zone. These tissue characteristics are associated with increased signal intensity within the transitional zone. The thin calcified zone is the deepest layer that divides the articular cartilage from the underlying subchondral bone. Generally, increased signal intensity (SI) is present within the transitional layers of cartilage, whereas decreased SI can be found within the deepest cartilage layer nearest the tidemark and subchondral plate (see Fig. 3.1 ).

Optimal MRI pulse sequences for cartilage evaluation should provide differential contrast between synovial fluid, fibrocartilage and articular cartilage, as well as sufficiently high voxel resolution. High image resolution is required for accurate assessment and reliable of cartilage lesions with MRI. Guidelines established by the Osteoarthritis Research Society International (OARSI) recommend that voxels be no larger than 0.5 by 0.5 by 1.5 mm for reliable assessment of cartilage defects less than 0.5 mm 2 . In practice this means that a cartilage lesion must be at least twice the geometrical diagonal of the voxel size to be reliably and accurately detected. Ample resolution is particularly important for the evaluation of early degenerative changes related to the onset and progression of primary osteoarthritis (OA) and posttraumatic osteoarthritis (PTOA) because patterns of cartilage degeneration can vary from focal losses to diffuse thinning over a large area ( Fig. 3.2 ).

Multiplanar, high-resolution two-dimensional (2D) sequences are the clinical standard for the evaluation of cartilage pathological conditions; they have been validated against an arthroscopic standard and have been shown to be highly reproducible. , Three-dimensional (3D) sequences may provide increased sensitivity and specificity for detection of cartilage lesions because they allow for higher quality multiplanar reformations and partial volume signal averaging is decreased. , Intermediate (IM) and T2 weighted FSE sequences allow for high sensitivity and specificity in the detection of cartilage lesions , , and are often favoured clinically because generated images are versatile enough to be used for the evaluation of other knee joint structures (e.g., menisci, ligaments, bone marrow). Fat-suppressed, fluid-sensitive, IM weighted FSE sequences are also useful because they allow for differential contrast among high-SI fluid, intermediate-SI articular cartilage and low-SI menisci, tendons and ligaments ( Fig. 3.3 ; see Fig. 3.2 ).

MRI is well suited for the evaluation of pathological conditions of cartilage and can provide clinically useful information to inform surgical candidacy. Cartilage lesions can occur as the result of either degenerative or traumatic processes that carry characteristic differences in their corresponding MRI appearance (see Figs 3.2 and 3.3 ). Cartilage degeneration initially manifests as disorganisation of the collagen matrix, decreased PG content and increased water content. Conventional MRI sequences demonstrate these early degenerative changes as alterations in expected signal, with pathological cartilage exhibiting prolonged relaxation times and corresponding signal hyperintensities. Patients with early degenerative cartilage damage may also exhibit fibrillation, pitting or fissuring of the articular surface. , Later stages of cartilage disease include loss of chondral substance that manifests as both focal defects and changes in cartilage thickness. End-stage arthrosis involves multiple partial-thickness and full-thickness chondral defects, cartilage delamination and diffuse thinning (see Fig. 3.2 ). Patients with degenerative cartilage lesions often exhibit concomitant changes within the subchondral bone, including the formation of subchondral flattening, osteophytes, oedema, sclerosis and cysts. Traumatic cartilage injuries are the result of acute mechanical overloads that generate shearing or impaction damage to the articular surface. Shearing injuries typically result in partial- or full-thickness cartilage defects that may affect the underlying subchondral bone. Linear cartilage flap tears and delamination injuries can also occur as the result of shear force overload. Cartilage shearing injuries are often unstable and are often associated with displaced cartilaginous or osteochondral fragments (see Fig. 3.3 ). Unstable lesions are unlikely to heal without surgical intervention. Compressive and rotational overloads can result in cartilage impaction injuries that can manifest as focal signal hyperintensities, depression of the articular surface or trabecular fracture lines. Even in the absence of cartilage signal changes or gross defects, bone marrow oedema patterns serve as evidence of substantial forces transmitted thorough the cartilage at the time of injury and can help identify cartilage at risk for degenerative change ( Fig. 3.4 ). , Several morphological cartilage MRI scoring systems are available and can provide a semiquantitative metric for the evaluation of lesions within the cartilage and surrounding tissues. Modified Noyes and Outerbridge scoring systems, the Whole-Organ MRI Score (WORMS) and the MR Imaging Osteoarthritis Knee Score (MOAKS) are among some of the most commonly used. , Research has also focused on quantitative evaluations of joint morphology, including measurements of cartilage thickness and volume. , These techniques require high field strength (3T), high voxel resolution and precise segmentation of cartilage and subchondral bone surfaces. Cartilage thickness measurements are favoured over volumetric measurements for longitudinal evaluation of the cartilage because thickness measurements have greater sensitivity to regional changes that may be overlooked if evaluating volumetric measurements exclusively. , , ,

Meniscus
Medial and lateral menisci are fibrocartilaginous structures attached to tibial surface and serve to transmit tibiofemoral compressive loads, as well as provide increased geometrical conformity (mechanical stability) to the tibiofemoral joint. More than 50% of load in the lateral compartment is transmitted through the lateral meniscus, whereas the medial meniscus transmits approximately 50% of the medial compartment load. , Menisci are formed from a dense extracellular matrix (ECM) composed of primarily water (≈70%) and collagen (≈20%), with PGs and other proteins composing the remaining dry weight. , Normal menisci measure 3 to 5 mm in height. The medial meniscus is semicircular in shape with an anterior horn that is less broad (6 mm) than its posterior horn (12 mm), whereas the lateral meniscus is nearly circular, with a uniform width (10 mm) from anterior to posterior. , ,
Normal meniscal tissue appears with relatively homogeneous hypointense signal on all conventional MRI sequences. Low SI within the intact meniscus can be attributed to its highly organised fibrocartilaginous structure that works to constrain protons and promote spin-spin interactions, leading to rapid spin dephasing and shortening of T2 relaxation. Sagittal plane MR images are typically used to evaluate anatomy and pathological conditions within anterior and posterior meniscal horns, whereas coronal images are well suited for evaluation of the meniscal bodies and the root attachments. Depending on the through plane resolution and the thickness (or absence) of an interslice gap, axial plane images can also be used to confirm circumferential tear patterns by providing direct visualisation of the entire meniscal surface and free edge adjacent to the tibial spine. IM or PD weighted MR images are recommended for the evaluation of the menisci because they are sensitive to the decreases in collagen organisation and the imbibition of synovial fluid caused by tears or degeneration. As with the evaluation of articular cartilage, adequate voxel resolution is essential for accurate and reliable detection of defects and other pathological conditions. Use of PD weighted FSE pulse sequences is therefore advantageous because they allow for faster data acquisition and thus make it possible to achieve better image resolution within a clinically feasible scan time.
Partial meniscectomy after meniscal injury is the most common orthopaedic surgical procedure performed in the United States. Regardless of surgical or nonsurgical treatment, meniscal tears lead to degenerative joint changes and are associated with a fourfold increase in the risk of developing knee OA. , , Damage to the meniscus results in disorganisation of the collagen fibres and subsequent increases in mobile water content. Synovial fluid is imbibed by the meniscus, resulting increased SI within areas of pathological defects. Tears within the medial meniscus typically originate on the inferior surface of the posterior horn, whereas the lateral meniscus is predisposed to transverse or oblique tears.
To classify the range of meniscal pathologies, an MR grading system based on signal distribution relative to an articular meniscal surface or meniscal apex can be used. Grade 1 and grade 2 meniscal pathological conditions involve intrasubstance degeneration with an absence of gross defects. Specifically, a grade 1 meniscal pathological condition can be defined as a nonarticular focal or globular intrasubstance increase in SI that is usually the result of mechanical loading or degeneration. However, grade 1 changes may not be clinically significant because these changes are often apparent in asymptomatic athletes and normal volunteers. , Grade 2 meniscal lesions are defined as horizontal, linear intrasubstance increases in SI that do not extend to a meniscal articular surface. Grade 3 meniscal lesions are gross meniscal tears that can be identified by linear signal hyperintensity crossing the meniscal substance and extending to at least one articular surface, with or without changes in gross meniscal morphology (extrusion). In the absence of prior surgery, there is a positive predictive value for meniscal tear of 95% if increased intrasubstance signal extending to the articular surface or morphological distortion of the meniscus is present on two or more consecutive MR images.
Typically, meniscal tears can be described as either acute or degenerative and are classified by their orientation relative to the meniscal body ( Figs 3.4 to 3.6 ; see Fig. 3.2 ). Acute (traumatic) tears have a specific incidence of injury and are the result of excessive forces applied to normal meniscal tissue, whereas degenerative tears (see Fig. 3.2 ) are the result of normal repetitive forces that produce degeneration over time. Although most meniscal tears can be visualised in sagittal plane MR images, coronal and axial plane images can be important for accurate assessment and characterisation of various tear patterns. , Accurate characterisation of meniscal tear pattern is critical for proper clinical management because some tears are more amenable to surgical repair than others.


Horizontal tears extend in a predominately horizontal course across the meniscal body, separating the meniscus into superior and inferior sections (see Fig. 3.2B ). These tears are often degenerative and typically occur in patients older than age 40. , On MRI, horizontal tears demonstrate linear hyperintensity that extends to the free edge of the meniscus in a course approximately parallel to the tibial plateau. When they occur in younger patients, they are not uncommonly associated with meniscal cysts and may manifest as a primary soft tissue mass at the joint line.
Vertical tears extend to the meniscal surface as either longitudinal or radial tears. Longitudinal tears run perpendicular to the tibial plateau and divide the central and peripheral portions of the meniscus. Unlike horizontal and radial tears, pure longitudinal tears do not involve the free edge of the meniscus. MR images of longitudinal tears exhibit a vertically oriented line of high SI through the meniscus that contacts at least one of the articular surfaces. Longitudinal tears typically occur as the result of trauma and are common in younger patients who have sustained a significant knee injury such as anterior cruciate ligament (ACL) rupture. In fact, 90% of medial and 83% of lateral peripheral longitudinal tears are associated with an ACL injury. , Careful assessment of the location of the tear relative to the vascular supply of the meniscus should be undertaken; tears located in the peripheral third are amenable to primary repair as opposed to those location in the inner margin of the meniscus closer to the intercondylar notch.
Radial tears are vertical tears that are oriented perpendicular to the free edge of the meniscus and can occur as either ‘classic’ radial tears or root tears (see Figs 3.4 and 3.6 ). Classic radial tears are more common in the lateral meniscus, whereas root tears are more commonly observed in the posterior horn of the medial meniscus. The latter tears are common in the presence of OA and may be manifest acutely as accompanying bone marrow oedema pattern in the subjacent tibia. Displaced flap tears may also occur in this location and, depending on their chronicity, may be asymptomatic. Classic radial tears are not often repaired because they often involve the avascular ‘white zone’ of the meniscus and thus have a poor chance of healing. However, acute root tears are often amenable to repair because the surrounding synovial blood supply facilitates healing. Both classic radial tears and root tears result in the disruption of meniscal hoop strength, which can manifest as peripheral subluxation or partial extrusion of the meniscal body. The appearance of radial tears on MR images varies by the location of pathological conditions and the imaging plane used. A radial tear within the meniscal body can manifest as a cleft on sagittal plane MR images, whereas the same tear would appear as a truncated or ‘ghost’ meniscus on coronal plane images. In contrast, a radial tear through the meniscal horn can appear as a truncated or ‘ghost’ meniscus on sagittal plane images, whereas coronal plane images depict the same tear as a cleft. ,
Bucket handle tears are longitudinally oriented vertical tears with the ‘handle’ fragment composed of a centrally migrated inner (torn) portion ( Fig. 3.7 ; see Figs 3.5 and 3.6 ). Because these tears often result in mechanical obstruction that can generate persistent pain and locking, surgical repair or debridement can be required. Bucket handle tears are seven times more likely to occur in the medial meniscus compared with the lateral meniscus. A double posterior cruciate ligament (PCL) sign is often evident on sagittal plane images, with the displaced bucket handle fragment sitting parallel to the PCL (see Figs 3.5A and 3.7 ). Other imaging signs that can indicate the presence of this tear pattern include an absent bow tie appearance, a fragment within the intercondylar notch, a ‘double’ anterior horn (flipped meniscus) and an abnormally small posterior horn. The location of bucket handle tears relative to the vascular periphery of the meniscus, and the integrity of the ‘handle’, dictate the potential for successful surgical repair.

Tendon and Ligament
Like the meniscus, tendons and ligaments have highly ordered collagen ultrastructures that work to constrain protons (e.g., increase spin-spin interactions) and result in the characteristic hypointense signal appreciated on conventional MRI sequences. , Exceptions to this are the ACL, which demonstrates a striated appearance as a result of interspersed bands of fat and the orientation and thickness of its collagen bundles, and the distal quadriceps tendon where fibro-fatty connective tissue found between tendon layers produces longitudinal streaks of intermediate signal. Tendons connect muscles to bone and are responsible for transmitting forces between these two structures to facilitate joint motion. Ligaments connect bone to bone and serve to provide joint stability and guide joint motion. Injury to tendons and ligaments can be classified on a scale from 1 to 3. Grade 1 tendon and ligament injuries are mild with no gross abnormalities present and manifest on conventional MRI series as hyperintense signal and thickening of the fibres, often with some adjacent soft tissue oedema. Grade 2 injuries are indicative of partial tears and are demonstrated on MRI with signal changes of greater magnitude than seen in mild injuries, with partial tears present within the tissue. Grade 3 injuries represent a complete rupture of the tendon or ligament; MRI images demonstrate signal changes associated with significant oedema extending into the adjacent soft tissues and lack of tendon or ligament continuity, with an obvious fluid-filled gap between the stump fibres that will attenuate or resorb with time.
Anterior and posterior cruciate ligaments
ACL and PCL are static stabilisers to the knee joint that provide not only anteroposterior stability but also assist the collateral ligaments in maintaining rotational stability. Imaging acquisition protocols for the evaluation of morphology and SI of the ACL and PCL are identical. Acquisition of IM weighted FSE and T2 weighted fluid-sensitive FSE sequences in all three planes (axial, sagittal, coronal) is typically used for evaluation of the cruciate ligaments.
ACL tissue is composed of two distinct bundles, the anteromedial bundle (AMB) and the posterolateral bundle (PLB). The AMB is slightly larger and is tauter in flexion compared with the PLB, whereas the PLB is taut in extension. , Depending on the scan parameters, it can be difficult to distinguish between the two ACL bundles in the sagittal plane; however, they can be differentiated readily using axial and coronal plane sequences. At the time of MRI acquisition, the knee is typically in extension and the normal ACL will appear as a 3- to 4-mm-thick band with a taut anterior margin and a Blumensaat angle of 15 degrees or less ( Fig. 3.8A ). , Because the ACL is composed of densely packed bundles of collagen fibres encased in loose connective tissue, it exhibits low SI, separated by linear stripes of intermediate to bright SI on non–fat-suppressed sequences. These striations within the ACL are indicative of the interposition of fat and synovium that exists between the collagen fascicles. , Although all three imaging planes are routinely used to evaluate the ACL, sagittal image sets are the primary plane for evaluation of the ACL because they best demonstrate the femoral and tibial attachments (see Fig. 3.8A ).

MR evaluation of the ACL has high sensitivity and specificity for the identification of ACL ruptures and can provide valuable clinical information in the event of concomitant injury that may require additional intervention. , Ruptures of the ACL are evident as gross disruption of the ligament, with the remaining fibres demonstrating a hyperintense, thickened appearance ( Fig. 3.8B ). Because ACL ruptures are commonly associated with a pivot shift mechanism of injury, transchondral impaction injuries are often evident over the anterolateral femoral condyle and the posterolateral tibial plateau. These impaction injuries manifest as focal areas of oedema within the subchondral bone on fluid-sensitive, fat-suppressed images ( Fig. 3.9 ) and may also result in gross cartilage damage (see Fig. 3.3 ). Cartilage on the condyles is more subject to compression and the tibial plateau cartilage is more subject to shear as the tibia translates and rotates. Bone marrow oedema patterns tend to be absent in the setting of partial ACL injuries. Partial ACL tears are more difficult to diagnose than complete ruptures , and are delineated by signal hyperintensity within the ligament, a thickening of fibres and potential changes in overall ligament morphology with horizontal orientation of a band, particularly in the subacute to more chronic setting. Chronic ACL tears lack the oedema and haemorrhage seen with acute ACL injuries, and when viewed in the sagittal plane, the ACL typically lacks a normal slope parallel to the Blumensaat line. Chronically torn ACLs can appear with low SI throughout (after scar remodelling), atrophic or resorbed (absent).

The PCL courses from the medial femur to the posterior middle tibia; it is typically less than 6 mm in the anteroposterior dimension and is nearly twice as thick as the ACL. , , On all conventional pulse sequences, the PCL appears as homogeneously hypointense and lacks the intrasubstance fat that gives the ACL its striated appearance. PCL tears account for approximately 3% of all knee injuries and are more commonly associated with multiligamentous injuries. Unlike the ACL injuries, damage to the PCL often does not result in gross disruption of the ligament but rather the ligament stretches significantly and becomes structurally incompetent. , Thickening of the PCL to more than 7 mm increases the clinical suspicion for a tear, with a sensitivity of 94% and specificity of 92%.
Medial collateral ligament
The medial collateral ligament (MCL) is composed of both superficial and deep fibres and measures approximately 1.5 cm in the anteroposterior dimension. The superficial component originates on the medial femoral condyle at the adductor tubercle and inserts distally on the tibia deep to the pes anserine tendon insertions approximately 5 to 7 cm below the joint line. , The deep fibres are composed of the meniscofemoral ligament, which is attached to the femur distal and deep to the superficial fibres, as well as the meniscotibial ligament, which is attached to the medial tibial articular cartilage edge. Normal MCL tissue appears as a continuous band with low SI along the medial joint line.
MCL injuries occur as a result of valgus overload to the knee and are therefore commonly associated with concomitant lateral sided contusions, often at the posterior margin of the knee as a result of injury sustained in flexion. , Because of the deeper position and relatively short length, the deep component of the MCL is more at risk for injury compared with the superficial component. , , MRI evaluation of grade 3 MCL injuries (full-thickness ruptures) demonstrates abnormal intrasubstance signal and complete discontinuity of fibres. Avulsion of the MCL may occur at its proximal or distal attachments (though proximal is more common) and is often accompanied by bone marrow oedema patterns and injuries to the menisci or cruciate ligaments. MRI evaluation of chronic MCL injuries exhibits abnormal SI, ligament thickening and laxity or discontinuity of the fibres.
Extensor Mechanism
The three main components of the knee extensor mechanism are the patella, the quadriceps tendon and the patellar tendon. The patellar tendon originates on the inferior pole of the patella and inserts on the tibial tubercle and typically measures approximately 5 cm in length. As with other short T2 tissues, the patellar tendon is typically hypointense on conventional MRI sequences but may contain focal areas of increased SI along the posterior aspect of the proximal and distal ends. The distal quadriceps tendon receives contributions from the four muscles of the quadriceps muscle group that converge and insert on the superior patellar border: The rectus femoris constitutes the most anterior layer, whereas the vastus medialis and vastus lateralis contributions make up the middle layer, and the vastus intermedius forms the deepest layer. Although most of the fibres of the quadriceps tendon insert on the superior pole of the patella, some of its fibres course over the anterior aspect of the patella to form an aponeurosis that connects with the patellar tendon. At the patellar insertion, fibrofatty connective tissue is present between the aforementioned tendon layers, resulting in longitudinal streaks of intermediate signal within the otherwise hypointense quadriceps tendon ( Fig. 3.10 ). The patella is the largest sesamoid bone in the body; , it serves as a link between the patellar and quadriceps tendon and serves to facilitate extension by increasing the mechanical advantage of the quadriceps muscle by extending its effective moment arm. Typically, patellar length is similar to the length of the patellar tendon. , Patellar cartilage is the thickest cartilage in the body (4 to 6 mm), the lower third of which articulates with the femoral trochlear surface during MRI examinations when the knee is relaxed in extension. , Medially the patella exhibits a convex medial facet, whereas the lateral aspect demonstrates a concave lateral facet. Normally the lateral femoral condyle projects further into the anteroposterior dimension than the medial condyle and acts in conjunction with the medial retinaculum and medial patellofemoral ligament to prevent lateral dislocation of the patella. Sagittal and axial plane IM weighted FSE sequences paired with a fluid-sensitive T2 weighted acquisition provide optimal visualisation of patellar anatomy. MR images of the patella demonstrate signal intensities consistent with that of bone marrow, cortex and cartilage seen elsewhere.

Disorders of extensor mechanism are a common cause of anterior knee pain and can range in severity from tendinosis to gross patellar dislocations. Extensor tendinosis is relatively common and can predispose tendons to rupture. On MRI, tendinosis is demonstrated by signal hyperintensity within the tendon substance and a thickening of fibres; oedema within the adjacent bone marrow may also be present. Pathological patellar tendinosis findings are typically most conspicuous in the proximal posterior aspect of the tendon, whereas quadriceps tendinosis occurs at or near its insertion on the proximal aspect of the patella. Patellar tendon ruptures are relatively uncommon, affecting less than 0.5% of the population each year. , Complete disruption of the patellar tendon most commonly occurs as a proximal avulsion (with or without bone) from the inferior pole of the patella. , Acute tears of the quadriceps tendon are more common than patellar tendon ruptures and are typically observed at or just proximal to the superior pole of the patella. Quadriceps and patellar tendon ruptures are most often observed in older male patients with a history of tendinopathy. Complete ruptures of the patellar and quadriceps tendons exhibit an obvious fluid-filled gap between the retracted tendon and the patella or the stump fibres. Patellar maltracking and patellofemoral instability are associated with an increased incidence of patellar dislocation, impingement and OA and occur as a result of a combination of anatomical abnormalities that work to disrupt the balance of medial and lateral stabilisers about the patella. Impingement of the infrapatellar fat pad secondary to abnormal patellofemoral mechanics generates soft tissue oedema along the superolateral aspect of the fat pad on fat-suppressed, fluid-sensitive images. Risk factors for patellofemoral maltracking and instability include trochlear dysplasia, patella alta and a decreased lateral trochlear inclination angle, all of which are readily appreciated on MRI. Patients with trochlear dysplasia exhibit trochlea joint surfaces that are shallow or flattened proximally, with less evident concavity distally. MRI evaluation of trochlear dysplasia severity includes measurements of lateral trochlear inclination, trochlear facet asymmetry and trochlear depth, all of which are best appreciated on axial or sagittal images. , A lateral trochlear inclination angle of less than 11 degrees, trochlear facet ratio of less than 40% and trochlear depth 3 mm or less are all indicative of trochlear dysplasia. , Superoinferior patellar position is routinely measured using the Insall-Salvati ratio, in which the length of the patellar tendon is divided by the maximal diagonal length of the patella. On sagittal images, Insall-Salvati values greater than 1.3 are indicative of patella alta. Superoinferior patellar position can also be measured using the Caton-Deschamps index, in which the measured distance between the inferior osseous border of the patellar cartilage to the top of the tibial plateau is divided by the superoinferior length of the patellar articular cartilage surface. A normal Caton-Deschamps index falls between 0.6 and 1.3, whereas patella baja is defined by an index less than 0.6, and patella alta is indicated by a Caton-Deschamps index greater than 1.3. ,
Quantitative and Advanced MRI Techniques
Although it is clinically useful to evaluate tissue morphology with conventional MRI techniques, morphological assessments remain indirect measures of the biostructural integrity of tissues. Because tissue damage does not always manifest as gross morphological change, quantitative MRI (qMRI) metrics can be used as noninvasive means by which to monitor the onset and progression of disease, as well as inform treatment plans and evaluate intervention efficacy ( Table 3.2 ). qMRI techniques for the assessment of tissue biostructure have been established and work through the exploitation of inherent characteristics in MRI tissue relaxometry that reflect tissue histological characteristics and ultrastructural integrity. When using quantitative techniques, care must be taken to be cognisant of the normal relaxation times of the tissue under study. For example, using a pulse sequence intended for articular cartilage to the meniscus or ligament will not capture the shorter relaxation times of the latter tissues and will simply measure noise, rather than anything biologically meaningful.
MR Imaging Technique | Biostructural Property Evaluated | Strengths | Limitations |
---|---|---|---|
T2 mapping | Collagen orientation and water content | Well validated; does not require use of contrast agent; compatible with most MR systems and field strengths; no contrast agent required | Susceptible to magic angle effects; cannot evaluate calcified cartilage layer |
T2∗ mapping | Collagen orientation and water content | Can be faster than T2 mapping; UTE sequences allow for evaluation of calcified cartilage layer; no contrast agent required | Susceptible to magnetic field inhomogeneities and magic angle effects |
T1ρ mapping | PG/GAG content and distribution | Sensitive to early PG/GAG depletion; no contrast agent required | Optimal at 3T; not available across all institutions |
dGEMRIC | PG/GAG content and distribution | Well validated indirect measurement of PG/GAG content (high sensitivity and specificity) | Requires use of Gd contrast agent (contraindicated m pts with renal impairment); long delay (≈1.5 h) between Gd administration and postcontrast MRI |
ZTE MRI | Osseous detail/ features | CT-like contrast with MRI; can acquire high resolution images amenable to MPR; no ionising radiation exposure; no contrast agent required | Unable to achieve same resolution as CT within clinically reasonable time; not available across all institutions |
MAVRIC | Soft tissues in the presence of metal artefact | Allows for visualisation of soft tissues close to metal (previously obscured by artefact); no contrast agent required | Lower resolution and fixed number of bins with some software (time constraint); lack of slab or slice selectivity |
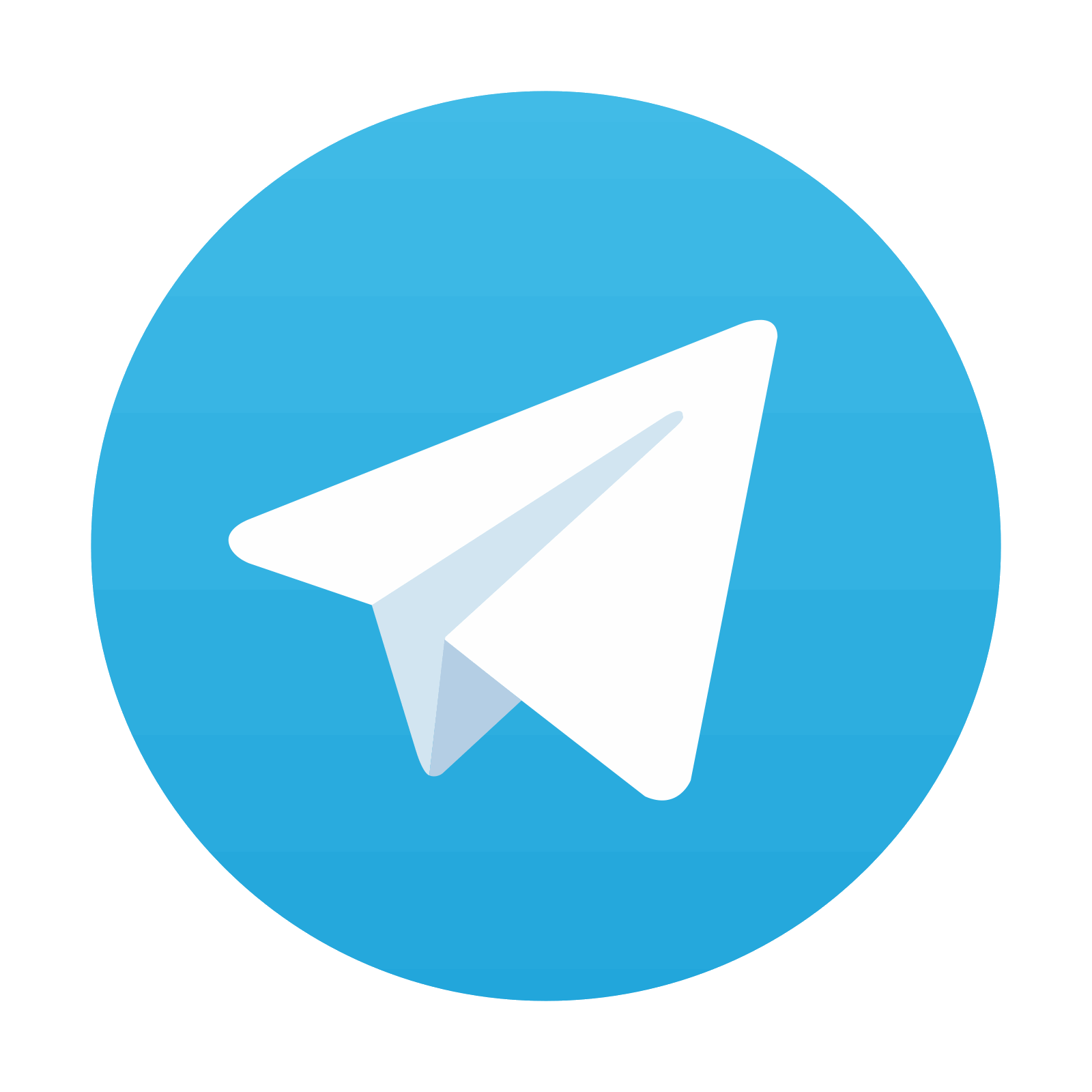
Stay updated, free articles. Join our Telegram channel
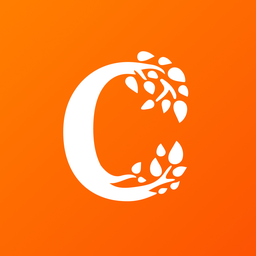
Full access? Get Clinical Tree
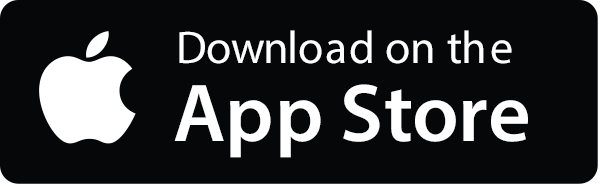
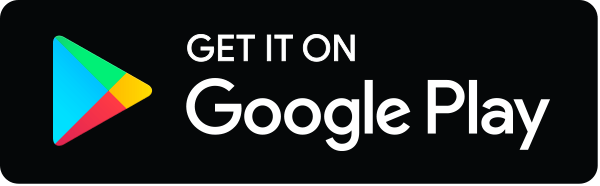
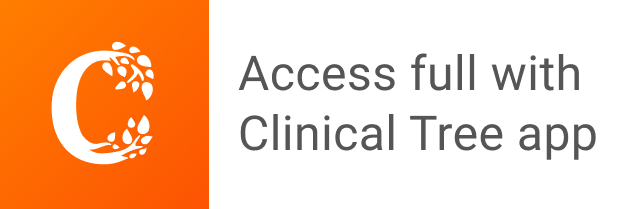