Knee Biomechanics and Implant Design
Timothy M. Wright, PhD
Fernando J. Quevedo Gonzalez, PhD
The mechanics and to a large extent the clinical performance of total knee arthroplasty can best be explained by considering design objectives. Replacing diseased and damaged tissues with human-made implants requires that the resulting composite structure restore normal joint function while also transferring large loads across the joint. The implant components themselves must remain well-fixed to the supporting bone and be as wear-resistant as possible to maintain a low-friction articulation and to avoid osteolysis. The challenge in designing an effective knee replacement is that these goals are competing; the optimal solution to reach one goal may be far from optimal to reach another. Ensuring normal joint kinematics, for example, requires articular surfaces that are not overly constrained. However, reduced constraint usually means reduced conformity between the surfaces, which may lead to unacceptably high contact stresses that increase the chance for wear and mechanical loosening of the implant components.
Contemporary knee replacement designs form an array of solutions aimed at reaching the best compromise between these competing objectives. Understanding how a specific design solution affects mechanical performance requires consideration of the functional and structural aspects of the normal knee joint, together with the mechanical principles that control kinematics, load transmission, and wear in a bone-implant system.
LESSONS FROM THE NATURAL KNEE JOINT
Functional Considerations
The primary motion of the knee joint is flexion and extension in the sagittal plane. The kinematics of the femur, tibia, and patella during this activity are determined by the anatomy of the femoral condyles, the tibial plateaus, and the patella; the muscle forces exerted across the joint; and the constraints provided by the cruciate and collateral ligaments. In the sagittal plane, the tibial plateaus are relatively flat, whereas the femoral condyles can each be approximated by two radii, a large radius forming the inferior portion of the condyle that contacts the plateau in extension and a smaller radius forming the posterior portion that contacts in flexion. If load transmission were based only on the bony anatomy, contact between the condyles and the plateaus would occur over a very small area, regardless of joint position, thus producing unacceptably large contact stresses. Fortunately, the menisci and the articular cartilage layers serve as effective load-transmission structures, distributing the joint loads over larger contact areas.
As the knee is flexed, the constraint provided by the posterior cruciate ligament (PCL) causes the femur to roll back on the tibia. The posterior translation combined with the smaller radii of curvature of the femoral condyles contacting the tibia as flexion increases provide the knee with a large range of motion. Rollback also increases the quadriceps moment arm by increasing the distance between the femorotibial contact points and the line of action of the muscle, providing a mechanical advantage for the muscle in resisting further flexion and in extending the knee.1
Rollback is asymmetric; femorotibial contact translates more posteriorly on the lateral than on the medial plateau, consistent with internal rotation of the tibia in flexion. The asymmetric rollback with greater translation of the lateral compartment of the knee can also be described as an internal rotation of the tibial relative to the femur. As the knee flexes, the tibia rotates internally guided by the anatomy of the condyles and forces exerted by the soft tissues. However, tibial rotation is quite variable. For example, in a recent study of 19 adult men between the ages of 45 and 75 years, the amount of internal rotation averaged between 11° and 12°, but the standard deviation was 6.6°.2 The same was true for other activities such as climbing and descending stairs, bowling, and golfing. Similarly, in a study combining fluoroscopy with computed tomography to determine the three-dimensional, in vivo, weight-bearing kinematics of five normal knees, wide variations in tibial rotation were found across several activities.3 For example, during rising from a chair, the average internal rotation was 19°, but the values ranged from 3° to 32° across the five subjects.
The primary motion of the patella with respect to the femur is in the sagittal plane. The kinematics of the natural patella is complex and affected markedly by the internal rotation of the tibia as the knee flexes. The bony anatomy of the patella and of the patellar groove of the femur provides considerable constraint to the patellofemoral joint,4 but during flexion, subject-specific anatomy
combines with soft tissue forces to cause the patella to rotate and translate about all three anatomic axes, including a medial shift by as much as 5 mm as the knee flexes.5,6,7 Abnormalities in the way that the patella tracks along the trochlear groove of the distal femur can be the cause of patellofemoral disorders such as pain, instability, and arthritis. The reporting of patellar tracking is affected significantly by how coordinate systems are defined and by the way in which the joint is loaded and moved during testing. The measurement accuracy is important as differences in tracking can be small. Comparison between existing studies is difficult because of differences in methodology. Nonetheless, other than general agreement that the patella translates medially in early knee flexion and then translates laterally, other motions are less consistent and highly variable.8
combines with soft tissue forces to cause the patella to rotate and translate about all three anatomic axes, including a medial shift by as much as 5 mm as the knee flexes.5,6,7 Abnormalities in the way that the patella tracks along the trochlear groove of the distal femur can be the cause of patellofemoral disorders such as pain, instability, and arthritis. The reporting of patellar tracking is affected significantly by how coordinate systems are defined and by the way in which the joint is loaded and moved during testing. The measurement accuracy is important as differences in tracking can be small. Comparison between existing studies is difficult because of differences in methodology. Nonetheless, other than general agreement that the patella translates medially in early knee flexion and then translates laterally, other motions are less consistent and highly variable.8
The total resultant force on the distal femur is made up of a component due to patellofemoral contact and a component due to tibiofemoral contact.1 During normal gait, the contact points between the patella and the femur and between the tibia and the femur move, and the positions and magnitudes of the contact forces change. However, the positions and magnitudes of these forces vary in such a way that the direction of the total resultant force on the tibia remains relatively constant. This is consistent with the need for only small variations in surface curvature of the tibial plateau to maintain equilibrium through joint compression loading.1
Knee motions in the medial-lateral plane and in internal and external rotation are considerably smaller than in flexion-extension. Varus and valgus moments are created across the knee joint because of the medial-lateral components of the ground reaction force that occur during daily activities such as walking (Fig. 9-1). The knee resists these external moments by redistributing the load transmitted between the two plateaus.1,9 Little medial-lateral translation is required between the joint surfaces; rather, the compliant nature of the meniscus, articular cartilage, and underlying subchondral bone provide the ability for load redistribution.
When the knee joint experiences varus or valgus moment, the pressure distribution on the two plateaus creates a net contact force acting on the tibia that is in reasonable alignment with the patellar ligament force (Fig. 9-2). But in response to a varus moment, for example, increased pressure is produced in the medial compartment of the knee, while pressure is reduced in the lateral compartment (Fig. 9-3); in effect, the location of the net contact force shifts to the medial side of the joint. The contact force creates an internal valgus moment about the center of the knee joint that resists the externally applied varus moment. Given the compliant nature of articular cartilage, the amount of joint angulation required to produce such redistribution in the pattern of surface deformation is small, typically less than a degree. Shifting the contact force as a mechanism for resisting varus and valgus moments has its limitations, of course, because the
contact force can shift only as far as the outer edge of the joint. For larger varus or valgus moments, such as when the line of action of the ground reaction force passes at a larger distance from the knee than in normal gait, supplementary mechanisms, such as additional muscle forces, are necessary to generate an adequate resisting moment. However, the moment arms of the muscles about the center of the knee are quite short; because of this mechanical disadvantage, large muscle forces are necessary (Fig. 9-4).
contact force can shift only as far as the outer edge of the joint. For larger varus or valgus moments, such as when the line of action of the ground reaction force passes at a larger distance from the knee than in normal gait, supplementary mechanisms, such as additional muscle forces, are necessary to generate an adequate resisting moment. However, the moment arms of the muscles about the center of the knee are quite short; because of this mechanical disadvantage, large muscle forces are necessary (Fig. 9-4).
![]() FIGURE 9-3 When the ground reaction force passes medially from the knee (the same conditions as shown in Fig. 9-1), the resulting varus moment can be resisted by the valgus moment created by a shift of the contact force to the medial side of the joint. (Adapted from Burstein AH, Wright TM. Fundamentals of Orthopaedic Biomechanics. Baltimore: Williams & Wilkins; 1994, Fig. 3-15.) |
![]() FIGURE 9-4 When the ground reaction force passes a greater distance from the knee (than is shown in Fig. 9-3), additional muscle forces act along with the contact force to offset the varus moment. (Adapted from Burstein AH, Wright TM. Fundamentals of Orthopaedic Biomechanics. Baltimore: Williams & Wilkins; 1994, Fig. 3-16.) |
With extreme externally applied moments, such as might occur in athletic activities or during a traumatic event, redistribution of joint pressures and additional muscle forces are insufficient to generate a large enough internal resisting moment. In such circumstances, a large angulation of the knee results in lift-off of one condyle and stretching of the collateral ligaments (Fig. 9-5). Such extreme conditions place considerable mechanical burden on the knee joint.
The mechanical disadvantage of the muscle and joint contact forces about the knee in resisting externally applied functional loads creates both large muscle forces and large contact forces. Such loads cannot be measured directly in normal knees but loads across the tibiofemoral joint have been measured with instrumented total knee devices implanted in patients. The recorded values of joint load in these patients reached as high as four times body weight between the femur and tibia during normal activities of daily living.9,10 Large contact forces also occur across the patellofemoral joint. In activities such as stair climbing, a ground reaction force of body weight passes far away from the knee joint (Fig. 9-6). To offset the resulting flexion moment, the quadriceps muscle must provide a much larger force than body weight to maintain equilibrium and control joint position. Patellofemoral contact forces exceed three times body weight for these more strenuous activities, but also exceed one and a half times body weight even for level walking.11,12 In general, contact pressures increase with flexion angle and increase as the quadriceps angle (Q angle) deviates from normal.9
Structural Considerations
Transmission of the joint contact loads into the supporting cancellous bone and then to the metaphyseal cortex dominates the mechanical burden placed on the knee joint. In comparison to these large loads, the additional ligamentous loads placed on the joint are quite small. Consider the tibia, for example. Below the cartilage surface and subchondral bone, the joint loads are borne primarily by cancellous bone, which is quite dense under the tibial plateaus, decreasing distally (Fig. 9-7). The denser the cancellous bone, the greater its stiffness and strength,13 so the greater its load-carrying capacity. Therefore, the density distribution of the cancellous bone provides a map of the load-transmission pathway through the epiphysis.
While the cancellous bone density decreases distally, the density and thickness of the cortical bone forming the outer shell of the proximal tibia increase. The shell is thin and porous near the joint line, with mechanical properties more like that of the cancellous bone in this region,14 but the shell becomes thicker and denser (and thus stiffer) as the metaphysis is approached. The relative portions of the load carried by the two structures (the cancellous bone and the outer shell) are determined by their relative stiffness. The structure that has the greater stiffness carries more of the load (this concept is explored in more detail later for the case of a knee joint with a total joint replacement). Thus, the axial load is carried almost entirely by the cancellous bone near the joint and is gradually transmitted to the cortical bone as the stiffness of the cancellous bone decreases and the stiffness of the outer shell increases.
Cancellous bone density and hence its structural mechanical properties also vary with position in the transverse plane of the proximal tibia.15 The greatest density occurs beneath the central plateaus, the regions in contact with the femoral condyles during most activities of daily living. The lowest density bone is found in the region between the plateaus. This bone has little stiffness or strength, consistent with the low mechanical demand required by this region. Density also varies in the anteroposterior direction, with denser bone often present in the posterior portions of the plateaus, especially on the lateral condyle.15
For the distal femur, the cancellous bone is most dense near the joint, immediately beneath the articular surfaces of the condyles, and in the region of the trochlear groove. Both the distribution of density and the orientation of the cancellous bone architecture in the distal femur show that the load is transferred from the dense subchondral bone to the cortical bone of the diaphysis over a relatively short metaphyseal region.16 As with the tibia, the cortical shell is quite thin near the joint and so adds little to load transmission.
KNEE REPLACEMENT DESIGN
In restoring function by reconstructing a diseased or damaged knee joint, the implant designer must seek adequate kinematics and joint stability while maximizing the implant’s longevity in terms of maintaining fixation of the implant components to the surrounding bone and limiting damage and wear to the implant components. Just as the bony anatomy of the natural knee and the mechanical properties of the soft tissues directly control the kinematics, stability, contact forces, and load transmission across the joint, the designer’s choices of geometries and materials for the implant determine these same biomechanical traits for the knee replacement. The requirements for adequate function while also achieving adequate long-term fixation and damage resistance can create conflicting design objectives. To make rational choices among knee replacement systems, the surgeon must understand the interplay between competing requirements for total knee replacements and the influence of the implant’s design factors on each of these functional requirements.
Most modern total knee replacements consist of a metallic femoral component with a bicondylar convex articular surface that replaces the femoral condyles, a tibial component consisting of a metallic tray and a polyethylene insert to replace the tibial plateaus, and a polyethylene component to resurface the patella. Conceptually speaking, we can divide the process of knee replacement design into (1) the design of the articular surfaces, which determines the kinematics, joint stability, and wear characteristics of the implant and (2) the design of the fixation features, which determines how the load is transferred from the implant to the surrounding bone.
Design of Articular Surfaces
When designing the articular surfaces, the designer must balance the competing objectives of achieving adequate kinematics, like that in the natural knee, ensuring adequate joint stability, and generating low-contact stresses to reduce polyethylene damage and wear.
Kinematics
Knee replacement designs control their main motion, flexion-extension, primarily through the geometry of the femoral condyles and the tibial plateaus in the sagittal plane. Most contemporary total knee designs approximate the anteroposterior geometry of the natural femoral condyles using two radii: a large radius that contacts the plateau near extension and a smaller posterior radius that contacts as the knee flexes. Also known as J-curve, this design differs from single-radius designs that utilize a unique radius of curvature for the femoral condyles in the sagittal plane. Single-radius designs were introduced to improve mid-flexion instability (instability between 30° and 45° of flexion) and to provide a longer extensor moment arm, thus reducing quadriceps forces during extension.17 Clinical results with single versus J-curve type designs are equivocal with randomized clinical trials that show no difference in clinical or functional outcomes18,19 and others that show improved outcomes for single-radius designs.20,21
The articular geometry of the tibial surface is concave, usually with a larger radius than that of the femoral condyles. The concave shape forces the femoral component to rest in the lowest or most distal point of the tibial articular surface, called the dwell point. However, the larger radius of the tibial surface provides some laxity in the anteroposterior direction, allowing the femoral component to roll back on the tibial component as the knee flexes. In this way, the closer the radius of the femoral and tibial surfaces, the lesser the anteroposterior translation of the femur. Deep-dish (also called ultracongruent) designs are an example of how designers have exploited such radial conformity to constraint anteroposterior translation of the femur by more conforming tibial and femoral surfaces.
The rollback of the femur on the tibia as the knee flexes is ensured by one of two methods. The first method is retention of the PCL so that it provides the same function as in the natural knee; these implants are known as cruciate-retaining (CR) designs. CR designs often use relatively flat surfaces on the plateaus of the tibial component so that the articular surfaces do not constrain the posterior translation created by the pull of the ligament. Flatter surfaces are advantageous for contact stresses when load is transferred through both condyles, but they are at disadvantage when varus-valgus rotations are introduced, as edge loading may occur. Advantages of PCL retention include more natural kinematics and maintenance of the proprioception and load transfer capabilities of the ligament.22,23 However, the PCL is difficult to balance at surgery and often does not remain functional after knee replacement.24,25 Furthermore, studies showed that proprioception did not differ whether the ligament was retained or not at knee replacement.26,27 To ensure proper PCL function after knee replacement, the joint line must remain near its preoperative level or kinematics will be adversely affected. For example, if too thick a tibial component is used, the joint line will be raised, and the PCL will become tight in flexion, adding to the femorotibial contact force and increasing contact stresses and polyethylene wear.28
Alternatively, the PCL ligament can be replaced by a geometric constraint to anterior translation of the femoral component through a post-and-cam mechanism; these implants are known as posterior-stabilized (PS) designs. In PS designs, posterior translation of the femur as the knee flexes is ensured by combining a posteriorly positioned dwell point with the post-cam mechanism (Fig. 9-8). Before the femoral cam engages the tibial post, the femoral component sits at the dwell point and shows little posterior translation as the knee flexes provided that large compressive loads are applied across the joint, such as would occur during level gait. After post-cam engagement, the cam “pushes” on the post, resulting in posterior rollback of the femur. The flexion angle at which the post and cam engage depends on several factors, including the shape of the tibial and femoral articular surfaces, the surgical placement and orientation of the knee components, and the variations in knee loading among patients and across activities of daily living. Reports from in vivo kinematic studies of PS patients showed that cam-post engagement does indeed vary considerably from as little as about 30° of flexion to as much as 90°.29,30
Substituting for the PCL provides both range of motion and joint stability and allows for more conforming surfaces without compromising kinematics.31 Posterior stabilized designs have disadvantages as well. Greater bone must be resected from the femoral intercondylar notch than in CR designs to make room for the cam mechanism. Furthermore, significant wear and even fracture of the polyethylene post can occur because of impingement of the post with the femoral component.32,33
Clinical performance and outcome differences between CR and PS designs are generally insignificant. Meta-analysis of randomized clinical trials and comprehensive review of studies directly comparing CR and PS designs have found few differences,34,35 though both types of studies revealed greater range of motion with PS designs. This difference is likely the result of the controlled rollback that is afforded by the post-cam mechanism, as has been shown in in vivo fluoroscopic analyses of patients with CR and PS total knee replacements (Fig. 9-9).36
High-flexion versions of CR and PS designs incorporate design changes to accommodate flexion typically beyond 135°. These changes include decreasing the femoral condyle radii at mid- and high-flexion, reducing the anterior-central portion of the tibial plateau to avoid patellofemoral impingement and, in the case of PS designs, changes to the cam and post to avoid dislocation as the cam moves toward the top of the intercondylar spine. However, as with CR versus PS designs, meta-analyses of randomized clinical trials revealed that high-flex knee replacements did not appear to confer any benefit as compared to standard designs.37,38
Flexion-extension in the natural knee is accompanied by rotational motion in the axial plane. For knee replacements, combining curved surfaces in the anteroposterior direction with curvature in the medial-lateral direction (creating a toroidal shape) is a common method used to provide rotational laxity to knee replacements.39 Rotational laxity is limited even in the natural knee, so these curved surfaces can be quite, although not completely, conforming in the medial-lateral direction40 and nonetheless allow sufficient axial rotation. Many designs use a single radius for each condyle of the femoral component and a single, slightly larger radius for each tibial plateau; however, toroidal geometries have the added advantage of being somewhat forgiving in terms of surgical positioning and orientation. Even if the components are not ideally positioned and oriented with respect to one another, contact between toroidal surfaces provides contact between curved surfaces and hence larger contact areas.
Many current designs use the same two radii in the lateral and medial condyles so that the implant is symmetric about the sagittal plane. However, alterations from symmetric condylar geometries are sometimes included to use the femorotibial surfaces to guide internal rotation of the tibia. The prime example of this approach is the medial pivot design, which forces contact to remain near the center of the medial plateau by incorporating a large, single degree-of-freedom, ball and socket articulation, combined with a much less conforming lateral plateau. More recently, CR designs have employed asymmetric geometries with the lateral distal radius extended posteriorly to further aid natural anteroposterior rollback when the PCL is present.
The other main design approach to guide internal rotation of the tibia is that of mobile-bearing knee replacements. In these designs, the bearing needed for function is separate from the bearing between the articular surfaces.
The former bearing is typically placed between the tibial component and a polished metallic base plate, whereas the latter remains a more conventional bearing between the femoral and tibial components. The mobile nature of the tibial component, whether as a meniscal type bearing, a medial pivot bearing, or a rotating platform, is intended to allow the muscles and ligaments to control and constrain joint motion. Theoretically, this approach provides for normal joint kinematics while also allowing the articular joint surfaces to be more conforming than in a fixed bearing knee, leading to larger contact areas, lower contact stresses, and presumably better wear resistance.41,42,43 However, long-term clinical studies with results beyond 10 years of follow-up have found no difference between mobile- and fixed-bearing designs.44,45 Similarly, a longer-term study (with follow-up from 15 to 18 years) in young active bilateral total knee patients who had received a mobile-bearing design in one knee and a fixed-bearing in the contralateral knee found no superiority of the mobile-bearing total knee prosthesis over the fixed-bearing total knee prosthesis.46
The former bearing is typically placed between the tibial component and a polished metallic base plate, whereas the latter remains a more conventional bearing between the femoral and tibial components. The mobile nature of the tibial component, whether as a meniscal type bearing, a medial pivot bearing, or a rotating platform, is intended to allow the muscles and ligaments to control and constrain joint motion. Theoretically, this approach provides for normal joint kinematics while also allowing the articular joint surfaces to be more conforming than in a fixed bearing knee, leading to larger contact areas, lower contact stresses, and presumably better wear resistance.41,42,43 However, long-term clinical studies with results beyond 10 years of follow-up have found no difference between mobile- and fixed-bearing designs.44,45 Similarly, a longer-term study (with follow-up from 15 to 18 years) in young active bilateral total knee patients who had received a mobile-bearing design in one knee and a fixed-bearing in the contralateral knee found no superiority of the mobile-bearing total knee prosthesis over the fixed-bearing total knee prosthesis.46
The kinematics of the patellofemoral joint is an important part of any total knee replacement design. The need for a large range of motion leads to the same design dilemma as in the femorotibial joint: the need for articular geometries that cause decreased contact areas with flexion coincident with increasing contact forces. Patellar component design alone cannot be expected to provide the necessary constraint for all patients and all activities. Moreover, as with the natural knee joint, the stability of the patellofemoral joint depends on the constraint (or stability) of the femorotibial joint in a total knee replacement. Three general design solutions exist for patellar implants: an anatomic shape intended to provide a conforming patellar track; a spherical, dome shape for the patellar implant intended to eliminate the importance of rotational alignment between the patellar and femoral components; and a mobile bearing intended to have the same rotational advantage as the dome design but with more conforming articular surfaces.
Few well-controlled clinical studies exist to determine the effect of the patella design on clinical outcome. An in vivo fluoroscopic study compared the patella kinematics of normal volunteers and total knee patients performing a weight-bearing deep-knee bend from full knee extension to maximum flexion.47 The total knee patients had received either a dome-shaped or anatomic-shaped patella component. Both patellar component designs exhibited kinematics close to those of the normal patella, with good tracking of the patella in the trochlear groove throughout flexion. Though the patellofemoral contact areas were smaller in the total knee groups than the in the normal group, they were comparable between the two designs.
The kinematics of domed and anatomic patellar components have also been evaluated with computational models in combination with in vivo measurements.48 Twenty total knee patients, half with domed and half with anatomic patellar components, but all with the same femoral and tibial component design, performed two tasks—while the motions of the patella were tracked using stereo radiography. Subject-specific finite element models were used to combine the in vivo kinematics with the models to evaluate the effect of patella design on loading and kinematics of the quadriceps mechanism. Though the anatomic design demonstrated kinematics closer to that of natural knees, patient variability and compensation strategies seemed to mask the effect of implant design on functional performance.
Closely matching normal anatomy would appear an obvious goal to maintaining normal function after knee replacement, but reaching this goal intraoperatively relies on surgical skill to achieve both appropriate alignment between the three joint components and adequate soft tissue balance throughout the range of motion. Replacing normal anatomy with a dome-shaped patellar implant bearing against a concave femoral flange does not require the same level of anatomic reconstruction because the curved shapes allow a small amount of surgical and functional misalignment while maintaining curved surfaces in contact with curved surfaces. The primary disadvantage is in flexion in which contact becomes much less conforming, increasing contact stresses in the polyethylene of the patellar implant.49 Mobile-bearing designs allow very conforming geometries, thus addressing the contact problem.50 But they require a metal backing that reduces the maximum polyethylene thickness, leading to the potential for excessive wear and loosening (as discussed further in Contact Problems and Implant Wear).
Joint Stability
When adequate ligamentous constraint is present, such as in the case of many primary knee replacements, the designer can emphasize functional (i.e., kinematic) over joint stability (i.e., constraint) requirements. This is the case for designs such as the CR and PS described above, which can be considered low-constraint designs. However, knee replacement systems usually provide multiple options to the surgeon to address the situations of inadequate collateral ligaments and bony deformities, such as might be encountered at difficult primary and many revision surgeries. These implants must provide greater joint stability than a conventional knee replacement. The additional levels of constraint are often gathered under common classifications—PS-plus (or PS constrained), constrained condylar knees, and hinges— even though levels of constraint can differ among the offerings from device manufacturers within any one classification. Increasing constraint between the femoral and tibial component often comes at the cost of higher burden placed in the cement and surrounding bone. As we will describe later, higher constraint usually requires the use of stems to provide additional resistance to varus-valgus moments.51,52
PS-plus designs include small modifications to conventional PS designs to increase primarily the varus-valgus constraint. Such modifications often include a wider intercondylar spine, narrower femoral box, or more pronounced lips on the edges of the tibial articulation. Constrained condylar knee replacements provide constraint by a polyethylene central spine on the tibial insert that fits into a mating intercondylar box on the femoral component.53 The polyethylene spine and the metallic intercondylar box are more conforming than in a PS or PS-plus implant, such that varus-valgus and rotational motions are constrained when contact occurs between the medial and lateral surfaces of the spine and the corresponding surfaces of the box.
Mechanical laboratory testing has been used to examine limitations in the performance of these designs.54 A robot testing system was used to determine the joint stability in human cadaveric knees as described by the moment versus angular rotation behavior under varus-valgus moments through a range of flexion up to 90°.55 The primary stabilizing mechanism was found to be the redistribution of the contact force on the bearing surfaces (Fig. 9-10). Contact between the tibial post and the femoral box provided a secondary stabilizing mechanism after lift-off of a condyle had occurred. Collateral ligaments provide limited stability because little ligament elongation occurred under such small angular rotations. Compressive loads applied across the knee joint, such as would occur with the application of muscle forces, enhanced the ability of the bearing surfaces to provide resisting internal varus-valgus moment and, thus, reduced the exposure of the tibial post to the external varus-valgus loads.
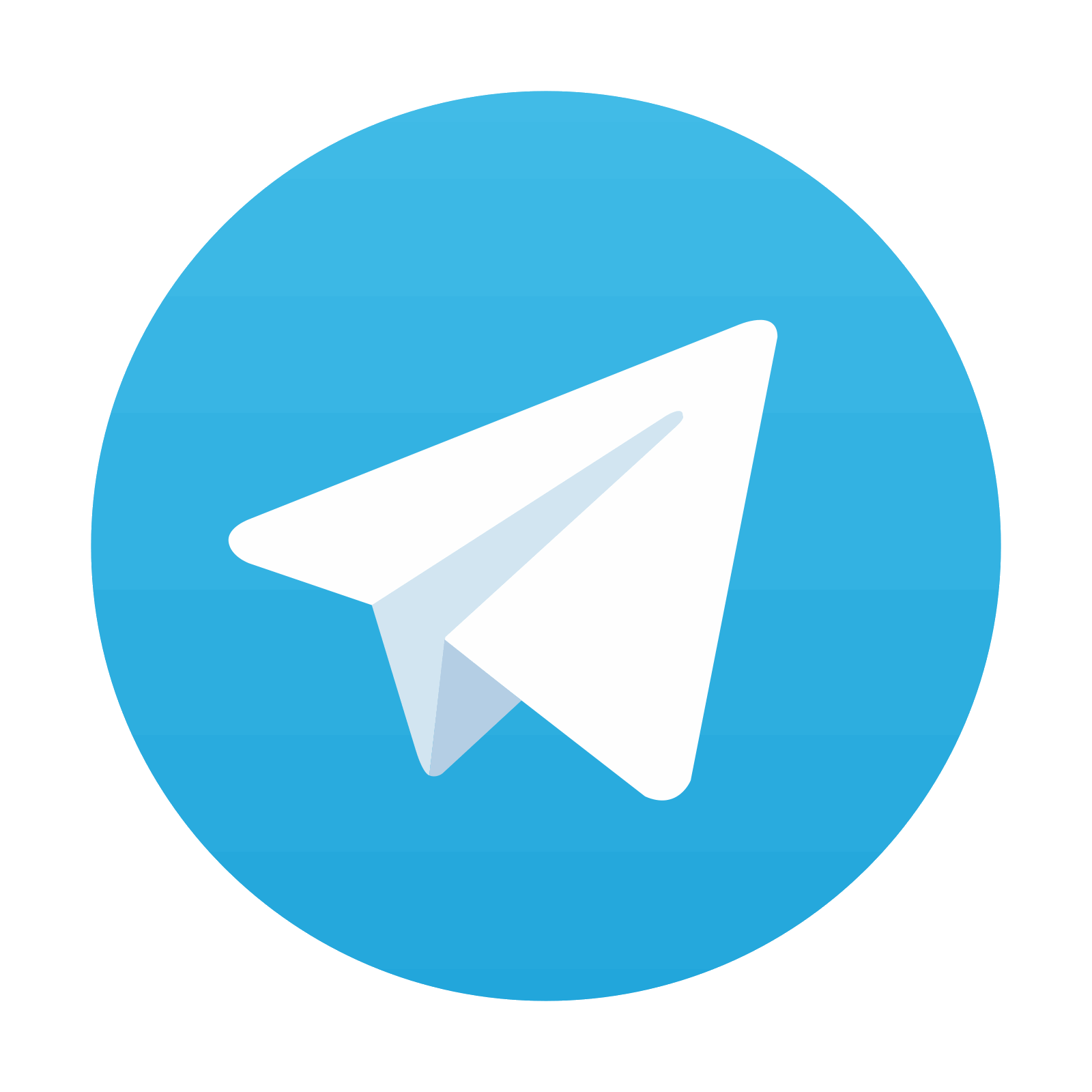
Stay updated, free articles. Join our Telegram channel
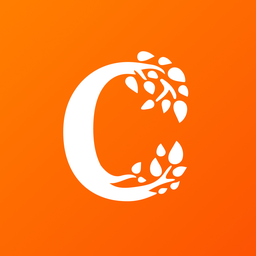
Full access? Get Clinical Tree
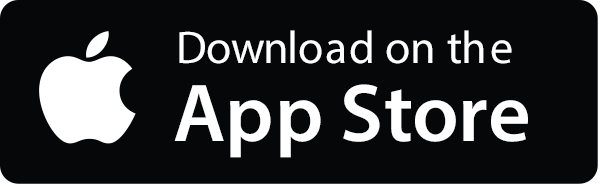
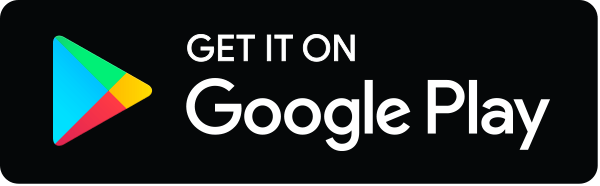
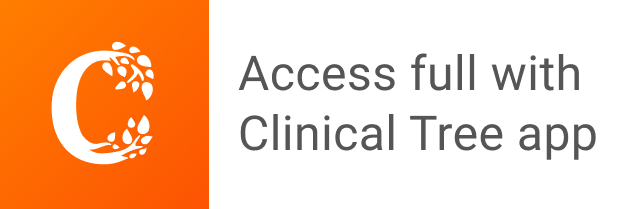