Interventional orthopedics is a developing field that attempts to bridge the gap between traditional non-operative orthopedics (e.g., sports medicine, interventional spine or pain medicine) and surgical interventions. This field expands the traditional approach to orthopedic problems, broadening the number of diagnoses and pathology that can be targeted with minimally invasive injections and procedures. For instance, instead of only evaluating orthopedic pathology as severe enough versus not severe enough for surgery, we offer alternative interventions for patients that have not responded to conservative therapy such as patients with partial tendon or ligament tears, ligament laxity, and nerve entrapment syndromes where surgical options are limited. The use of diagnostic ultrasound to complement the traditional orthopedic history and examination allows the clinician to more accurately diagnose and then target the underlying soft tissue and joint pathology.
Instead of traditional interventions being limited to unguided injections and surgery, interventional orthopedics utilizes interventional musculoskeletal ultrasound and fluoroscopy to guide injections to expand treatment options with the goal of precisely targeting and treating common orthopedic problems. The use of image guidance for procedures has increased over the past decades, largely driven by decreased equipment costs, patient safety initiatives, and higher-resolution imaging. In many cases, “blind” injections have been supplanted by image guidance, which gives the clinician the ability to directly visualize the target tissues and more accurately target specific pathology.
Therapeutic injections may include corticosteroids, but there is a focus on understanding the appropriate role of alternative injectates, which can be utilized to more accurately address the underlying pathophysiology. With the advent and expansion of regenerative treatments and orthobiologics, there is an increasing emphasis on tissue preservation, restoration of tissue function, and healing rather than solely procedures that target “inflammation” and only provide temporary pain relief, or more invasive surgical procedures carrying increased cost and risk of complications.
The traditional approach to the management of musculoskeletal pathology has largely been driven by locating and treating the primary pain generator. A good example is the treatment of low back pain. Typically, the interventionalist would try and identify a primary pain (i.e., the nerve root, facet joint, sacroiliac joint dysfunction, myofascial pain, or intradiscal pathology) and construct a treatment plan to specifically address the area of the spine most likely responsible for the patient symptoms. Conversely, an interventional orthopedics approach would take an approach of addressing the entire spine as a “functional spinal unit” and consider the interplay of these structures and the biomechanical role of adjacent ligaments, tendons, and muscles. The overall goal extends beyond general pain management and looks to address the underlying etiology of musculoskeletal pathology for long-term improvements in functional outcomes. With this in mind, the treatment plan for low back pain may include treating the lumbar facets, corresponding level epidurals if there is myoneural dysfunction on examination (e.g., weakness or gluteal enthesopathy at the posterior iliac crest), supraspinous and interspinous ligaments for stability, and possibly the multifidus muscle if there is decreased activation on examination and atrophy on magnetic resonance imaging (MRI).
The convergence of advances in imaging, an evolving understanding of the pathophysiology of both acute and chronic degenerative pathology, and a growing interest in minimally invasive approaches to orthopedic pathology has fueled this field and has expanded the type of injections and procedures performed. Some of the procedures discussed in this text did not exist before the widespread adoption of ultrasound. Many of these new procedures have become more common, including nerve hydrodissection, barbotage of calcific tendinosis, and percutaneous needle tenotomy procedures. Others are characterized by using specialized surgical tools or devices to duplicate surgical procedures using a percutaneous approach that will expand and continue to be adopted due to improved safety and morbidity.
The growth of regenerative injections, including but not limited to dextrose, platelet-rich plasma, and autologous stem cells, has also driven the emergence of new techniques and procedures. In some cases, the use of these treatments clinically has outpaced the scientific data. The scientific literature will undoubtedly evolve, and the field of interventional orthopedics will continue to mature and as we explore alternatives to many of the more traditional injectates and many surgical techniques that have limited evidence and efficacy. , Several studies have been published that question whether nonsurgical conservative measures, sham surgeries, or placebo therapy is as effective as management. In some cases, it is unclear if the traditional injections with corticosteroids or surgical interventions are better than non-operative management, placebo, or sham surgery, including the intermediate and long-term benefit of corticosteroids, arthroscopic meniscectomy, and debridement in patients with arthritis, or subacromial decompression surgery for rotator cuff impingement.
This introductory chapter focuses on the composition and organization of different tissue types and the current concepts in the pathophysiology of orthopedic conditions and how our understanding of common musculoskeletal conditions has influenced current and future management strategies. Conventional nonoperative therapies have targeted inflammation, but inflammation is important to the healing process. Treatment strategies must be tailored to the underlying tissue involved (nerve, muscle, tendon, ligament, bone, and cartilage) and the underlying pathology.
Tendinopathy
Tendons come in various shapes and sizes and connect muscle to bone. The normal tendon structure is largely composed of collagen and proteoglycans. Type I collagen comprises approximately 65% to 80% of the dry mass of the tendon, with smaller amounts of type II, III, IV, V, IX, and X collagen also present. Collagen molecules are cross-linked polypeptide chains, and their principal role is to resist tension, while proteoglycans are primarily responsible for the viscoelastic behavior of the tendon. The tendon is organized in a helical architecture, comparable to man-made ropes. This helical organization of the tendon components is present at various levels or organizations, including when collagen fibers are bundled together to form fascicles, and fascicles are bundled to form the tendon itself.
The cellular component of the tendon is made up of tenoblasts and tenocytes arranged in parallel rows among the collagen fibers. Tenoblasts are immature tendon cells and transform into tenocytes as they mature. Tenocytes function to synthesize collagen and other components of the extracellular matrix (ECM). Tenoblasts and tenocytes comprise 90% of the cellular component of the tendon, with the remaining 5% to 10% made up of chondrocytes, synovial cells, and vascular cells. ,
A thin film of loose connective tissue (endotenon) is present between the fascicles, allowing the fascicles to slide independently against each other. The endotenon is continuous with the connective tissue (epitenon) that surrounds the tendon as a whole ( Fig. 1.1 ). Some tendons, such as the Achilles tendon, have a paratenon that surrounds the tendon but separate from the tendon itself. The paratenon is made up of type I and III collagen fibers, and the inner surface is lined by synovial cells. In some cases, the tendon is surrounded by a true synovial sheath. There is often great confusion when describing the tissue that surrounds the tendon.

The tendon inserts on bone in the form of a myo-enthesis or cartilaginous entheses. Myo-enthesis have superior blood supply and are less prone to degenerative pathology. Intrinsic blood supply to the tendon is located at the myotendinous and osteotendinous junction, with extrinsic blood supply coming from the paratenon and synovial sheath. The musculotendinous junctions and entheses are vulnerable sites, and increased age and mechanical loading can decrease vascular supply to these areas. Small afferent nerves throughout the paratenon form plexuses with penetrating branches innervating the tendon.
Areas of the tendon with poor blood supply are at increased risk of injury. While tendon injuries can occur in the mid-tendon (i.e., Achilles), most pathology and pain arise at the enthesis. Poor blood supply predisposes damaged tendons to tissue hypoxia. Tendinopathy is thought to develop from excessive loading and tensile strain. Although load is a major component in the development pathology, the etiology of tendinopathy is likely multifactorial and includes genetics, age, body composition, comorbidities (e.g., dyslipidemias, rheumatoid disease, tumors, infections, heritable connective tissue diseases, endocrinopathies including thyroid disease, metabolic diseases including diabetes), and medication exposure (e.g., statin, fluoroquinolones).
The interplay between structural change, dysfunction, and pain is still not fully understood. Historically, tendon pain has been described as tendinitis, implying that inflammation was the central pathologic process. At the cellular level in early and chronic tendinopathy, there are an increased number of leukocytes (primarily macrophages and mast cells). However, compared to rheumatoid arthritis and other immune-driven pathology, the number of leukocytes is small, and there has been widespread recognition that the terminology of tendinitis, tendinosis, and paratenonitis should reflect the histopathologic feature of the tendon.
Histopathologic studies have shown the progression from normal ECM to reactive response and tendon disrepair, characterized by greater tissue matrix breakdown, collagen separation, neovascularization, and proliferation of abnormal tenocytes. The new model of tendon pathology is of a continuum that has three stages: reactive tendinopathy, tendon disrepair (failed healing), and degenerative tendinopathy. While these are described as three distinct stages for convenience, the idea of a continuum recognizes that the tendon can move forward or back along this continuum. This model highlights the need to tailor treatments to the specific tendon pathology and that a single intervention is unlikely to be efficacious in every case.
Ligament Injury
Similar to tendon tissue, ligaments are constructed from dense regular connective tissue and can vary in size, form, orientation, and location. Skeletal ligaments stabilize the joint and guide the joint through a normal range of motion and provide proprioception to coordinate movements. , The orientation of collagen fibrils tends to be in the direction of applied force, and while tendon collagen fibrils tend to be in parallel, the ligament collagen fibrils are not uniformly oriented as forces are applied in more than one direction. Type I collagen makes up 85% of the ligament, depending on the type of ligament, with the rest of the ligament composed of type III, VI,V, XI, and XIV collagen. Collagen bundles within ligaments have a crimped appearance, and with stress, the ligament elongates as collagen fibers uncrimp. This allows the ligament to elongate without sustaining damage, contributing to the viscoelastic property of the ligament.
In both tendons and ligaments, the major cell type is the fibroblast, or ligamentoblast and ligamentocytes. Epiligamentous plexus forms a net-like branching anastomotic pattern on the surface of the ligament with branches that penetrate the ligament and become intraligamentous vessels distributed into longitudinal channels within the ligament. The distribution of blood vessels varies among ligaments, and compared to the synovial tissue or bone, ligaments appear to be relatively hypovascular.
Ligaments are most often injured in traumatic injuries and follow the three phases of healing (inflammation, proliferative, and remodeling). Although the ligament may heal, the scar tissue that forms has major differences in collagen types, failure of collagen crosslinking, altered cell connections, small collagen fibril diameter, and increased vascularity. Even after fully healing, the ligament matrix apparels grossly, histologically, and biomechanically different from normal ligament tissue. The remodeled ligament can contain material other than collagen, including blood vessels, adipose cells, and inflammatory cells, resulting in weakness. , , In studies of injured medial collateral ligaments (MCLs), the ligament typically remains weaker after healing and only regains 40% to 80% of the strength and stiffness compared to normal MCLs. , The viscoelastic property of an injured ligament has a somewhat better recovery, returning to within 10% to 20% of normal.
Ligaments have a poor regenerative capacity due to the low cell density and lack of blood flow, and after an injury, the tissue is weaker, disorganized, and prone to reinjury. These persistent collagen abnormalities can present as symptoms of instability, with 7% to 42% of subjects reporting symptoms even 1 year after injury. Early resumption of activity can stimulate repair and restoration of function, while prolonged rest and immobilization delay or adversely affect recovery. In chronic instability, traditional treatment strategies, including immobilization, rest, nonsteroidal antiinflammatory drugs (NSAIDs), and corticosteroid injections fail to address the underlying pathophysiology. In vitro studies have shown platelet-rich plasma (PRP) induces proliferation of fibroblasts and the production on type I collagen, and there has been interest in the use of orthobiologics in the regeneration of ligaments.
Cartilage Injury
There are two common types of cartilage: hyaline and fibrocartilage. Hyaline cartilage is present at the connection between the ribs and the sternum, in the trachea, and on the articular surfaces of synovial joints. Hyaline cartilage is composed of a rich ground substance, glycosaminoglycans (GAGs), and collagen fibers (mainly type II collagen). Unlike most tissues, articular cartilage is devoid of blood vessels, nerves, or lymphatics. Fibrocartilage is present in intervertebral discs and meniscal tissue.
Hyaline Cartilage
Articular cartilage is hyaline cartilage within synovial joints and functions as a shock-absorbing tissue that provides low friction movement during articulation. Chondrocytes are sparsely distributed throughout the dense ECM of the articular cartilage, and the ECM is primarily composed of collagen, proteoglycans, and water ( Fig. 1.2 ). The composition of the ECM varies within different zones of the articular cartilage, and articular cartilage is typically divided into four zones: superficial, middle, deep, and calcified ( Table 1.1 ).

Zone | Cell | Thickness | Function |
---|---|---|---|
Superficial (STZ) |
| 10%–20% | Resists tensile forces and protects deeper layers from shear stresses |
Middle |
| 40%–60% | Resists compressive forces |
Deep |
| 30% | Resists compressive forces |
Calcified |
| Anchors collagen fibrils to the subchondral bone |
Injury to the articular cartilage can occur from a single traumatic event or repetitive microtrauma. Progressive cartilage injury can be accompanied by alteration in the underlying bone.
Articular cartilage has limited repair potential once damaged. In mature articular cartilage, chondrocytes are quiescent and no longer divide with very little turnover of the cartilage matrix. The articular cartilage receives nutrition mainly through diffusion from the synovial membrane and cyclic loading. The lack of a direct blood supply in articular cartilage, paucity of cells, and high matrix to cell ratio creates a challenging healing environment, and full-thickness articular cartilage defects rarely heal spontaneously. Treatment approaches for focal cartilage defects or osteochondral lesions vary, and there is no uniform approach. Techniques to treat focal cartilage defects are usually divided into marrow-stimulating (reparative) and reconstructive techniques.
Isolated lesions to the cartilage should be differentiated from osteoarthritis (OA), where there is more diffuse damage to the articular surface. While impacting the same tissue, the pathophysiology differs. OA is characterized by the involvement of the cartilage, synovial membrane, and subchondral bone, making OA a disease of the whole joint. The pathology is multifactorial but is driven by inflammatory mediators within the joint, resulting in pain, deformity, and loss of function.
The earlier changes in the cartilage often appear at the joint surface in areas where mechanical and shear stress are the greatest. In OA, chondrocytes go from being quiescent to becoming “activated,” characterized by cell proliferation, matrix degradation and remodeling, and inappropriate hypertrophy-like maturation. Degradation of the articular cartilage, thickening of the subchondral bone, osteophyte formation, and synovial inflammation. This proinflammatory environment can result in reduced chondrogenesis, as well as suppression of type II collagen synthesis. , These negative effects of inflammation on chondrogenic differentiation may have negative effects on cell-based therapy.
Treatment strategies for OA often involve behavioral (e.g., exercise and weight loss), pharmacologic (e.g., oral medications, injection therapy, and biologics), and in end stages, joint replacement surgery. Intra-articular injections are common in the management of OA; however, the dense articular cartilage is less permeable to injected medications penetrating the cartilage extracellular matrix, and the injectate can be rapidly cleared by the lymphatic system. , In recent years, there has been a growing interest in alternative approaches to injection therapy and altering joint homeostasis. There has been increased interest in the treatment of the subchondral bone in patients with OA and focal lesions. The proposed mechanism is stimulating subchondral bone that influences the articular cartilage because of communication and cross-talk between both tissues. ,
Fibrocartilage
Fibrocartilage contains high levels of type I and II collagen and is present between vertebral bodies, the pubic symphysis, menisci, labrum, and the tendon–bone interface.
Intervertebral Disc
The intervertebral discs’ major role is mechanical, transmitting load and forces through the spinal column. Structurally, the intervertebral disc is composed of the inner nucleus pulposus (NP) and an outer annulus fibrosus. The NP is gelatinous and primarily formed from water, proteoglycans, and randomly organized type II collagen, and the intrinsic hydrostatic pressure of the NP resists compressive loads. The outer fibrocartilaginous annulus fibrosus is composed of 15 to 25 concentric rings (lamellae), with elastin fibers and type I and II collagen fibers lying in parallel and provides the tensile strength of the disc.
With age, the nucleus generally becomes more fibrotic and less gel-like, and the collagen and elastin of the annular lamellae become irregular and disorganized. It can be challenging to differentiate changes that occur due to aging and those that might be “pathologic.” The most significant change that occurs in disc degeneration is the loss of proteoglycan (aggrecan), which is responsible for maintaining tissue hydration and impacts the disc load-bearing behavior. The collagen population of the disc also changes with degeneration, but these changes are not as obvious as those of the proteoglycans. , The loss of proteoglycan and matrix disorganization leads to an inability to maintain hydration, and when loaded, they lose height, bulge, and subsequently lead to inappropriate stress along the endplate or the annulus. , The loss of disc height can also affect adjacent structures, resulting in spinal stenosis, apophyseal joint arthropathy, and ligamentum flavum hypertrophy.
The intervertebral disc is largely avascular and must rely on passive diffusion from adjacent endplate vessels for nutrition. The limited vascular supply and indirect access to nutrition limit the discs’ intrinsic capacity for remodeling and repair. Traditional therapies may provide symptomatic relief but do not target the underlying degenerative pathophysiology. Newer cell-based therapies aim to achieve cellular repair.
Meniscus
Vascularization of the meniscus is from the lateral, medial, and middle genicular arteries, forming a perimeniscal capillary plexus. There is significant discrepancy in the vascularity of the meniscus, and the meniscus is often described as having three distinct zones characterized by the degree of vascularization. The outer zones with the greatest vascularity region are the red-red zone and the transitional red-white zone. The inner or central avascular zone is the white-white zone. The healing capacity is directly related to blood circulation, with the peripheral meniscus (red-red and red-white zone) having the greatest potential for healing. ,
The morphology of the meniscus cells also can be characterized by the zone in which the cells are found. There are three cell populations within the meniscus. The outer zone is mainly populated with fibroblast-like cells with an oval, fusiform shape and long cell extensions, which facilitate communication among cells and the extracellular matrix. These fibroblast-like cells are surrounded by dense connective tissue consisting of type I collagen, with a small percentage of glycoproteins and type III and V collagen present. The main cell type in the inner zone is classified as fibrochondrocytes or chondrocyte-like cells, and they have a chondrocyte appearance (round or oval-shaped). These fibrochondrocytes are embedded in a fibrocartilage matrix consisting mainly of type I (60%) and II (40%) collagen and aggrecan.
The superficial zone of the meniscus harbors progenitor cells.
Meniscal injuries are classified depending on location, thickness, and resulting instability. The type of tear has a significant impact on the ability of the tear to heal and the most appropriate and effective therapy. Partial or total meniscectomy can lead to altered loading dynamics, leading to degeneration and OA on an average of 14 years following surgery. , A detailed discussion of surgical management is beyond the scope of this chapter, but in general, there is an increased emphasis on meniscal preservation whenever possible to preserve loading dynamics in the knee. Tears in the vascular region do have the potential to heal due to the existing blood supply and the possibility of progenitor cells in this region.
Bone Pathology
The skeleton serves a variety of functions, providing support, permitting movement, and protecting vital internal organs. The skeleton also serves as a reservoir of hematopoietic stem cells, which give rise to blood cell lineages and mesenchymal stromal cells that are multipotent with the potential to differentiate into bone, cartilage, fat, or fibrous connective tissue. , In order to understand the mechanical properties of bone clinically, it is important to understand the component structure of bone.
At the macrostructure level, bone can be characterized as cancellous (trabecular) or cortical (compact) bone, with cortical bone forming a dense outer shell around the honeycomb-like structure of cancellous bone. Different bones have different ratios of cortical to cancellous bone. In long bones, the diaphysis is primarily composed of dense cortical bone, while the metaphysis and epiphysis are composed of cancellous bone surrounded by dense cortical bone. In general, cancellous bone is more metabolically active and remodeled more often than cortical bone. Bone is surrounded by an inner endosteal and an outer periosteal surface. The periosteum is a fibrous connective tissue sheath surrounding cortical bone and is tightly attached to the bone by thick collagenous fibers (Sharpey’s fibers). Unlike bone, the periosteum has nociceptive nerve endings and contains a store of bone-remodeling cells (osteoblasts) that play a role in healing fractures.
The microstructure of bone is highly complex. In cortical bone, large vascular channels (Haversian canals and Volkmann canals) are oriented along the longitudinal direction of the bone and contain the blood supply to compact bone. These channels are surrounded by compact highly mineralized cylindrical rings (lamellae). The lamellae and Haversian canal form the osteon or Haversian systems, which is the chief structural unit of cortical bone. Cancellous bone is spongy and fills the inside of many bones and has a rich vascular supply. ,
Regarding the nanostructures, the mineral content of bone is mostly tiny mineral crystals (calcium phosphate–based hydroxyapatite), which provide rigidity and load-bearing strength to bone. The organic matrix is primarily composed of collagenous proteins, which crosslink to add stability to the bone matrix. , Collagenous proteins compose 85% to 90% of bone proteins, with bone matrix mainly composed of type I collagen. ,
The primary cellular component of bone cells are osteocytes, osteoblasts, and osteoclasts. Osteoclasts are derived from a monocyte stem-cell lineage and carry out resorption of old bone, while osteoblasts are bone-forming cells and synthesize a new bone matrix. Osteoblasts are found in large numbers in the periosteum and endosteum, while osteocytes are osteoblasts that have become trapped in the calcified bone matrix. Together, osteoblasts and osteoclasts influence the remodeling of bone after trauma.
Bone adapts to physical stimuli, dietary changes, or injury. Bone is constantly undergoing remodeling to preserve bone strength. Remodeling occurs at sites that require repair but also occurs in a random manner throughout life. Woven bone is put down rapidly during growth or repair, with fibers aligned at random. As a result, woven bone has lower strength than lamellar bone, which has its fibers oriented in parallel and in line with the axis of stress.
Healing of cancellous bone with its rich vascular supply occurs more rapidly compared to the cortical bone that can be complicated by delay or nonunion. In normal fracture healing, osteoblasts form immature woven bone, resulting in early callus formation at the fracture margins. With remodeling, callus is replaced by lamellar bone. Delayed union is when healing is slower than anticipated, and a nonunion of a fracture is defined as a fracture where healing has not occurred at 9 months. There is a growing interest in orthobiologics for nonunion fractures, but there have been conflicting results in the literature. , Preclinical in vivo studies have suggested PRP may enhance bone regeneration with favorable results, but there are inherent limitations to the clinical translation of basic science studies and in the majority of studies PRP was used to augment surgery either at the time of surgery or a delayed injection. , Fewer studies have examined autologous stem cells in non-unions, and the real benefit of biologics for bone healing is unknown. ,
Unlike fractures that are generally classified by mechanism of injury (i.e. traumatic, pathologic, stress), the events leading to avascular necrosis (AVN) are incompletely understood, have unclear causality, and have delayed diagnoses. , Ischemia or direct toxic effects on bone marrow and cells may contribute to AVN, and necrosis predominantly develops at sites composed predominantly of adipocytes (yellow marrow), such as the femoral head. The natural history of AVN is better understood than the early triggering factors, with necrosis, inadequate remodeling, and eventually collapse of the necrotic segment and OA. Basic science and clinical trials of PRP may be more appropriate as an adjunct therapy, while autologous stem cells have shown promising results. ,
Nerve Injury
Nervous tissue consists of two types of cells, neurons and glial cells. Neurons are responsible for communication and are composed of the cell body (soma), dendrites, and axon. The dendrite receives information from other neurons, allowing the cell to integrate multiple impulses. Most cell bodies have multiple dendrites arising from the cell body, and dendrite-branching patterns are characteristic of each neuron. The axon arises from the cell body and propagates nerve impulses between cells, transporting nerve impulses along the axon, and the axon can branch repeatedly to communicate with many target cells. At the terminal end of the axon, synaptic junctions facilitate the transmission of the nerve impulse from one neuron to another or the target cell (muscle or gland cells) ( Fig. 1.3 ).
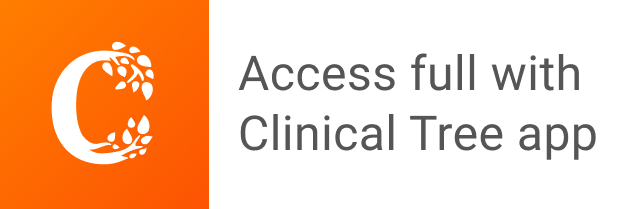