Key points
- •
The process of intervertebral disc (IVD) degeneration consists of gradual structural and biochemical changes that may lead to pain and disability.
- •
IVD degeneration is multifaceted, mediated by biomechanical alterations, nutritional compromise, genetics, and environmental factors among others
- •
Various imaging modalities exist to assess the IVD and degenerative phenotypes, whereas magnetic resonance imaging is considered the gold standard.
- •
Various classification schemes of IVD degeneration have been reported, whereby their clinical utility is constantly being assessed.
- •
Clinical management of symptomatic IVD changes remains tenuous; however, promising regenerative biologics have been developed and continue to be refined that may have clinical utility.
Intervertebral disc (IVD) degeneration is an age-related condition, often associated with many other clinical conditions such as spinal stenosis , disc prolapse, and low back pain (LBP) [ , ]. The intricate nature of IVD degeneration involving not only the IVD but the endplate and vertebral body makes it challenging to define; however, the term generally refers to the extensive morphological, metabolic, biochemical, and functional alterations of the IVD [ ]. IVD degeneration can occur at any age, but the risk increases with age [ ]. During the lifespan, one may experience such changes to a greater or lesser extent [ ].
The first and foremost known IVD alteration is reduced water and proteoglycan content, particularly in the nucleus pulposus (NP) (see Chapter 1 ). With advancing age, the size of the proteoglycan molecule decreases while the proportion of nonaggregated proteoglycans increases. Small fragment proteoglycans result in a dehydrated disc [ ]. The disc loses its hydrostatic ability, further compromising its functional role. Alternatively, an increase in cross-linking and thickening of the collagen fibers with age causes retention of the disc’s denatured collagen, which reduces the tissue’s strength [ ]. There are also altered metabolic activities as viable cellular density declines with age [ , ]. The spectrum of degeneration incorporates all these age-related changes [ ]. Signs of degeneration include an increase in fibrosity of the NP, an inward buckling of the inner annulus fibrosus (AF), appearance of circumferential and radial tears (responsible for radial bulge or herniation in many cases) in the AF, and a reduction in the IVD height [ ]. Such changes may be observed irrespective of age, gender, and spinal level. Additionally, these structural disruptions affect the IVD mechanical function, with depressurization of the NP and compressive stress concentrations in the AF (see Chapter 2 ) [ ]. This contributes to the build-up of stress gradients within the disc [ ]. Consequently, the stress response is the premature senescence of cells [ ] and the release of cytokines along with matrix-degrading enzymes [ ], leading to a perpetual cascade of IVD degeneration.
Degeneration and low back pain
The impact that IVD degeneration has on clinical symptomatology has long been an area of research interest. The global point prevalence of LBP has been estimated to be around 11.9% [ ], and the 1-year prevalence has been estimated to be between 22% and 65% [ ]. Moreover, out of 291 conditions assessed in the Global Burden of Disease 2010 study, LBP ranked first in disability and sixth in overall burden [ ]. Similarly, the global point prevalence of neck pain has been estimated to be between 5.9% and 22.2% [ ]. As populations continue to become older, the prevalence of LBP is likely to increase; in fact, by doing so, the impact that IVD degeneration has on pain potentially becomes even more important.
The association between IVD degeneration and LBP has been demonstrated in several large-scale cohort studies across diverse patient populations. Teraguchi et al. [ ] conducted a population-based analysis using the Wakayama Spine Cohort and assessed IVD degeneration and its relationship to pain in 975 adults. They found that lumbar IVD degeneration was significantly associated with LBP and that the severity of LBP increased with the number of IVDs affected. The TwinsUK study also found that the burden of IVD degeneration was a risk factor for episodes of LBP in a sample of over 1000 twins [ ]. De Schepper et al. [ ] assessed the relationship between LBP and IVD degeneration in 2819 adults aged 50 years or older from the Rotterdam Study. They found that IVD degeneration at two or more levels was strongly associated with LBP, although IVD degeneration at one level was also associated with LBP. These studies and others highlight the close relationship between LBP and IVD degeneration and suggest that the etiology of LBP may be discogenic in nature.
IVD degeneration has also been implicated in the development of LBP in younger populations. A study by Takatalo et al. [ ] assessed LBP in 554 young adults, with a mean age of 21 years, using the Northern Finland Birth Cohort 1986. They created five clusters of pain severity ranging from mostly asymptomatic to constantly in pain. In the three clusters with the highest degree of LBP, IVD degeneration was significantly more prevalent than their more asymptomatic counterparts. Furthermore, IVD degeneration was independently associated with pain, even when controlling for other degenerative findings. Furthermore, based on a study by Smith et al. [ ] assessing the Raine Study Cohort, 5 year trajectories of LBP were noted and strongly related to IVD degeneration and herniation at baseline. Another cross-sectional cohort study by Samartzis et al. [ ] assessed the prevalence of IVD degeneration in Southern Chinese in adolescent volunteers ranging from 13 to 20 years old. They found that IVD degeneration was present in 35% of volunteers and that IVD degeneration was significantly associated with the prevalence of LBP/sciatica, the intensity of LBP, diminished social functioning, and greater physical disability. Often IVD degeneration is thought of as a condition of the elderly, but adolescents and young adults can experience IVD degeneration-related LBP as well.
The relationship between cervical IVD degeneration and neck pain is still largely up for debate, although previous work has suggested a discogenic etiology of neck pain [ ]. The TwinsUK study found that while IVD degeneration contributed to neck pain, there was a larger component comprising psychosocial status [ ]. Conversely, in the aforementioned study by Teraguchi et al. [ ], no association between cervical IVD degeneration and neck pain was found. Some have suggested that degenerative findings in the absence of herniation or radiculopathy are not sufficient to cause neck pain [ ]. It is possible that the cervical and lumbar spine differ in their pain response to IVD degeneration, and it seems that overall while there is an association with neck pain, it is much less pronounced than in the lumbar spine.
Etiological factors of intervertebral disc degeneration
The etiology of IVD degeneration is multifaceted. In fact, despite decades of research, a clear picture of causation has not yet emerged but has become increasingly clear that an interaction effect between different causative factors and a personalized profile of risk and its variation therein is involved. It is essential to realize that there is no single key contributor to the process of IVD degeneration, but there are several factors that contribute to its pathogenesis. Some of the most important of these are genetic factors: a number of twin studies have shown that IVD is surprisingly heritable, with >70% of the phenotypic variation in IVD degeneration due to genetic factors [ ]. Determining what these genetic factors precisely are and how they act is altogether a greater challenge. While genetic factors may encode proteins critical to IVD metabolism or cell cycle, they may also be influential in driving or interacting with biomechanical, nutritional and/or body weight factors in addition to other pathways. Environmental factors and lifestyles are known to play a critical role in the initiation and progression of IVD degeneration.
Biomechanics
IVDs are “cushion”-like structures that support and evenly distribute the compressive and bending stresses acting upon the spine (see Chapters 1 and 2 ). Changes in the loading pattern, intensity, and duration of loading increase the risk of IVD [ , ]. Continuous and excessive static compression is believed to cause more damage to the disc than cyclic compression [ ]. Experiments performed on cadaveric motion segments have shown mechanical disc failure following prolonged compressive loading due to a break in the integrity of the endplate (see Chapter 10 ). Fracture of the adjacent endplate may be caused by a reduction in the hydrostatic pressure of the NP. Thereafter, the resistance to compressive force is mediated by the AF [ ], leading to the formation of stress peaks, particularly in the posterior AF [ ]. A nonsupporting NP gives way to the unstable inner AF, and it may buckle inwards [ , ], leading to disruption of the remaining tissue [ ]. The pressure gradients and stress peaks break the inter- and intralamellar connections of the annular fibers resulting in delamination and separation resulting in tears and fissures [ ].
It is suggested that the prolapse of the IVD might be a consequence of rotation and bending motions combined with compressive forces (see Chapter 8 ) [ , ]; these motions, when combined, are likely to produce radial fissures of the AF due to fatigue failure [ , ]. Torsion, another critical component of loading, is also thought to increase posterolateral stress leading to prolapse [ ]. The depressurized NP and fissured AF of the prolapsed IVD cannot resist the bending movement because there is an increase in the intralamellar shear stress, causing further mechanical disruption [ ].
All of these asymmetrical loading patterns, stress gradients, and tissue deformation are reported to affect the extracellular matrix and cellular organization of the IVD [ ]. Gene expression of both aggrecan and collagen II is downregulated [ , ], along with altered gene expression for specific matrix metalloproteinases (MMPs) and tissue inhibitors of metalloproteinases (TIMP) [ ]. Walter et al. [ ] reported mechanically induced cell death and a “shift toward catabolism” due to bending movements combined with compressive stress. IVDs with altered metabolism and diminished cells experience a chronic state of distress, leading to IVD degeneration [ ].
Altered loading patterns have been reproduced in many in vitro studies. Injuries to the IVDs and endplates of human cadaveric motion segments and in animal models were experimentally induced to determine their influence on IVD degeneration (see Chapters 3 and 4 ) [ , ]. The cut injury to the peripheral AF of sheep IVDs affirmed the role of tears and fissures in degeneration [ ]. Furthermore, human cadaveric studies helped demonstrate the role of endplate fracture in altering compressive load distribution in AF injuries [ ]. Reduction in the GAG content, upregulation of MMPs and cytokines, necrotic, and apoptotic cell death all are consequences of mechanically induced injuries to the endplate [ , ]. Similarly, a mechanically injured IVD causes upregulation of proteolytic enzymes and proteoglycan loss, releasing neurotrophins capable of promoting neuronal ingrowth and sensitization, which may link IVD degeneration and LBP [ ].
Nutrition
The avascular IVD deals with nutritional challenges throughout its life. Very few blood vessels and nerves are responsible for supplying the margins of the peripheral AF, while the rest of the IVD depends for nutritional support on capillaries and blood vessels present at the bone–cartilage endplate junction [ ]. The process of diffusion and fluid flow is mainly responsible for the transport of nutrients and waste through the endplate route [ ]. Oxygen and glucose are very crucial for the well-being of IVD cells and reach the disc tissue via the process of diffusion; metabolic waste such as lactic acid follows the same process but in the opposite direction [ ]. To and from transport occurs through the bony endplate and the dense matrix of cartilaginous endplates to reach the cells of the central IVD tissue [ ]. Any structural or biochemical change in the bony or cartilaginous endplate may influence the diffusion process [ ]. Multiple studies on endplates have linked the calcification and resorption of the cartilaginous endplate (CEP) with degeneration (see Chapter 10 ) [ , ]. Calcification and sclerosis of the bony endplate were also held responsible for IVD degeneration and the possibility that occlusion of the openings with capillary buds could deprive the IVD of nutrients [ ]. But, other studies incorporating precise quantification of bone mass density with the help of a μCT scanner showed no significant association of bony endplate sclerosis with IVD degeneration [ ]. Rather, an increase in porosity and permeability was seen with increasing IVD degeneration grades and with age [ ]. All of these findings draw attention toward the CEP, calcification of which may be a permeability barrier to the solute transport, facilitating the process of IVD degeneration [ ].
Other factors causing any disturbance in the nutritional supply to the IVD might be attributed to pathological changes in adjacent blood vessels through which nutrients diffuse. Lumbosacral spine segments are supplied by the branches of the abdominal aorta and internal iliac arteries, and thence atherosclerosis of the aorta or segmental arteries may compromise the nutrient supply to the IVDs [ , ]. Constriction of the blood capillaries due to smoking or short-term vibration could also be a significant factor in reducing the diffusional transport across the IVD [ ].
Oxygen and glucose are the main nutrients required by IVDs cells to stay alive. Glucose is chiefly used to provide energy, producing lactic acid as a byproduct in a sufficiently high quantity. The concentration of glucose is a sensitive parameter required to maintain the viability of the cell [ ]. With a disturbance in the transport across the IVD, the low levels of glucose and an increased accumulation of lactate compromise the viability of the cells. Reduced pH decreases the rate of matrix component synthesis and activates the production of proteases [ ]. A study using 3D finite element analysis recorded a reduction in the cellular density with nutritional deprivation [ ], hence, supporting the notion of nutrition-related mechanisms in IVD degeneration.
Genetics
Many studies provide extensive evidence of genetic factors playing a role in different IVD degeneration phenotypes. Family-based, twin and linkage studies, genome-wide association studies (GWAS), etc. have identified the genomic factors involved in IVD degeneration [ ]. Various phenotypes can be the subject of these studies, including the overall presence of IVD degeneration [ ], clinical sequelae, such as IVD herniation characterized by symptoms of sciatica [ , , ], or significant association of positive family history among other categorizations [ , ]. Gene–environment interactions are notoriously difficult to identify in any trait, including IVD ones. It is defined as “a different effect of environmental exposure on disease risk in persons with a gene mutation or, equivalently, a different effect of a gene on disease risk in persons with different environmental exposures” [ , ]. This concept leads to the notion that individuals with a greater genetic predisposition of IVD degeneration are more prone to respond to environmental factors that are possible but not proven. Among many features of IVD degeneration, IVD height, osteophytes (see Chapter 7 ), and annular bulging (see Chapter 8 ) are said to be heritable [ , ] and the progression of IVD degeneration is also genetically mediated (see Chapters 7 and 8 ) [ ].
Polymorphisms associated with the genes coding for known structural elements of the IVD have been studied using the candidate gene approach. Genes encoding collagen I (COL1A1) [ ], collagen IX (COL9A2 and COL9A3) [ , ], collagen XI (COL11A2) [ ], aggrecan [ , ], cartilage intermediate layer protein [ ], fibronectin [ ] and thrombospondins [ ], as well as genes coding for catabolic enzymes such as matrix metalloproteinases MMP3 [ , ], and inflammatory agents, such as interleukin 1 [ ], have been shown to be associated with IVD degeneration but lack robust replication. Several studies have also established an association with polymorphisms in genes coding for the vitamin D receptor [ , ] and growth differentiating factor 5 (GDF5) [ ] among others. The use of agnostic techniques, such as GWAS, which has been highly valuable in other traits including osteoarthritis, remains a powerful analytical tool for IVD degeneration genetic studies. Two studies have been published, one from Europe which identified the PARK2 gene on GWAS meta-analysis [ ] and the other from Asia which used a combined GWAS-linkage approach and revealed the CHST3 gene as associated with lumbar IVD degeneration [ ]. Of particular interest, neither group could replicate the others’ findings, suggesting an important influence of ethnicity on genetic associations. However, a recent study, by the GO Consortium initiative addressing approximately one million cases and controls identified variants in the CHST3 and SLC13A1 genes to be associated with more advanced forms of IVD degeneration, underscoring the implications of sulfate in disc pathology [ ]. Other limitations include different study designs, inclusion/exclusion criteria, sampling technique, limited analyses, and phenotype definition that weaken the level of evidence [ ]. Despite much work in the last 2 decades on the complex relationship between genetic factors and IVD degeneration, much remains to be done to identify the relevant genetic polymorphisms.
In the last decade, the role of noncoding RNA, including micro RNA (miRNA), has been identified as an important regulator of gene expression. Studies addressing IVD degeneration have confirmed that a variety miRNAs play a critical role in the apoptosis of NP cells, abnormal proliferation, production of inflammatory factors, degradation of the extracellular matrix and AF [ ]. The role of mitochondrial DNA dysfunction in the pathogenesis of disc degeneration has also been probed as mitochondria are important to maintain intervertebral disc homeostasis. It is revealed through different studies that mitochondrial dysfunction is able to promote IVD degeneration by inducing oxidative stress, mitophagy, apoptosis, and cellular senescence [ , ]. The pathogenesis, however, is still unclear and needs further exploration focusing on the molecular mechanisms of mitochondrial dysfunction [ ].
Additional risk factors of IVD
The risk of IVD degeneration has also been attributed to various environmental and lifestyle factors. Being overweight or obese, a smoker, physically inactive or overactive, or performing heavy manual labor may increase the risk of IVD degeneration [ , ]. Obesity is considered as an important factor for LBP [ ], and it is one of the most significant factors in IVD degeneration [ ]. A large population-based study by Samartzis et al. [ ] involving 2599 southern Chinese individuals demonstrated that increasing BMI was a risk factor for IVD degeneration. The authors also identified a positive association between BMI and a severity of IVD degeneration. Many studies have depicted the role of various adipokines in the process of IVD degeneration [ , ]. Adipokines, especially leptin, increase cellular proliferation [ ], alter cytoskeletal remodeling [ ], and release MMPs, hence, posing a high risk for the degenerative process [ ]. Furthermore, the excessive body mass of obese individuals exerts a high compressive load and may also shift the body’s center of mass anteriorly [ ] causing obese individuals to assume extreme lordotic posture [ ]. Hyperextension of the lumbar spine, as it occurs in the extreme lordotic posture, causes the concentration of compressive stress toward the posterior annulus, apophyseal joints, and between the spinous process (see Chapters 2 and 14 ) [ ]. Shear force is also believed to be raised at the L5 and S1 levels as the lordotic posture increases the inclination of the sacrum [ ]. Overweight and obesity are also associated with a decreased range of motion in the lumbar spine [ ]. This can gradually limit the functional capability of the spine [ ] and promote IVD degeneration.
Smoking has also been strongly linked to IVD degeneration [ , ]. Nicotine causes the production of carboxyhemoglobin and vasoconstriction, which in turn reduces the oxygen transport and blood supply. The constriction of capillaries surrounding the IVDs may impair nutritional transport and lead to degeneration [ ]. In vitro experiments on cell culture have shown reduced cell proliferation and synthesis of extracellular matrix, especially GAG [ ]. Moreover, the production of inflammatory cytokines following nicotine administration enhances the risk of degeneration [ ].
The potential association of some occupational hazards with IVD degeneration has also been emphasized by several studies but the effects are generally small [ , , ]. Heavy mechanical labor can lead to many spine-related pathologies including degeneration. Exposing the spine to frequent vibration may cause an accumulation of fatigue damage [ ]. The low metabolic environment of the IVD is not able to cope with the repetitive microscopic damage to the tissue.
The paraspinal muscles have also been implicated in their association with IVD degeneration and other spinal phenotypes [ ]. Variations in cross-sectional and fatty infiltration of paraspinal muscles have been noted between males and females, and age strata [ ]. The muscles have also been shown to be related to LBP [ , ]. See Chapter 15 for more elaboration on the implications of paraspinal muscles and spine changes as well as pain/disability.
Methods for assessing the phenotypes of disc degeneration
The association between IVD degeneration and LBP is now generally accepted [ , ]. For the past 3 decades, studies on animals and humans have encouraged refined assessment techniques for a better understanding of IVD degeneration. Multiple in vitro and in vivo methods have been reported to assess phenotypes of IVD degeneration in the lab and clinical settings [ ].
Direct visual inspection
It is not possible to visualize the IVD morphology with the naked eye in a clinical setting; however, a direct visual assessment of cadaveric specimens can provide some important information concerning the nature of lesions or defects that occur during life. Gross morphological examination of the IVD is useful in detecting very obvious features of disc degeneration. These include changes in the consistency of the NP, lamellar disorganization of the AF, the occurrence of tears and their types, and finally pigmentation of the IVD [ ]. IVD height loss, presence of osteophytes, and herniation are additional observations also indicative of IVD degeneration. Understanding the IVD morphology as it relates to different histological features in various injuries, inflicted [ ] or mechanical models [ , ], is a very common practice in preclinical studies (see Chapters 3 and 4 ). It is also applied to in vitro experiments using human cadaveric samples. The recently established role of the endplate in IVD degeneration has led scientists to look at this structure more closely [ , ]. Based on a large-scale study that macroscopically evaluated 1148 vertebral endplates, four different types of lesions, namely Schmorl’s nodes, calcification, fractures, and erosions were noted [ ]. Schmorl’s nodes were found to be the most common lesion. The second most common lesion was erosions, which appeared as a diffusive shallow breakdown of the endplate. Fractures of the endplate were also identified as clefts or fissures with irregular margins. Calcification of the vertebral endplate was the least common type of lesion [ ]. Calcification of the endplate was not associated with any structural damage or cracks of the endplate, but in life it may impede IVD nutrition by blocking the diffusion of nutrients [ ]. Exploring the link between endplate and IVD gross morphological changes with magnetic resonance imaging (MRI), if available, can help to clarify mechanisms of disc changes, their clinical relevance and potential targets for future therapies.
Gross morphological studies in animal and cadaveric tissues have been useful in determining overall IVD changes for academic research. These tissues are further analyzed at the microscopic level to study the mechanisms of disc degeneration.
Microscopy
Microscopy is an important and multidimensional in vitro technique that is very commonly practiced in a laboratory setup. The microscopic assessment provides information concerning soft tissue structure and organization. Such a level of detail can provide an important understanding, and in some cases may help inform future treatment strategies. Light microscopy with standard histological techniques is able to reveal many distinguishing features linked with the process of IVD degeneration. Many clinical and preclinical studies collect intervertebral disc tissues from patients after surgery, cadavers, and animals for histological staining and analysis. More specific information about the cellular and molecular composition of the IVD, inflammatory mediators, and the presence of blood vessels and nerves can be obtained by using immunohistochemical staining of specific molecules. The use of electron microscopy to identify normal or degenerate IVD ultrastructure [ ] and the recent introduction of wide-field fluorescence, laser scanning confocal microscopy, and 3D live cell imaging [ , ] have improved our ability to assess IVD. For a deeper and thorough understanding of the degenerative process, ultrastructural detail was studied under electron microscopy, focusing on the pattern of collagen fibrils and the cells of AF and NP. The use of fluorescent imaging [ , ] and confocal microscopy [ , ] have contributed to our understanding of various biochemical and inflammatory changes going on during the process of IVD degeneration. Differential interface contrast microscopy has also proved very effective in the study of structural organization [ ].
Plain radiographs
Plain radiographs are usually the first diagnostic choice of clinicians. It is applicable for the initial assessment for some spinal pathologies associated with LBP in some countries, especially if red flag signs are present. Plain radiographs are inexpensive and easily accessible. For general investigation of the spine, two radiographic views, anteroposterior and lateral, are taken to assess any fracture in the vertebra or endplate along with any congenital or acquired deformities of the spine. The additional findings from the radiographs include the possible presence of osteophytes, reduction of IVD height, and sclerosis of the endplate (see Chapters 7, 10, and 11 ). All of these are interpreted as signs of IVD degeneration [ , ]. With special reference to the vertebral endplate, morphological assessments involving an evaluation of endplate shape and size can also be done on plain radiographs but is unable to detect any IVD pathology and soft tissue abnormality.
Computed tomography
Computed tomography (CT) is beneficial for bony details and is capable of imaging certain conditions, such as herniation and spinal stenosis (see Chapter 13 ) [ ]. It can also visualize IVD morphology and, in combination with a myelogram, provides a detailed image of nerve roots that is essential in investigating the cause of LBP [ ]. However, this approach may not be universally routine clinical practice. In addition, CT discography is not considered as a very rational approach due to its invasive and painful nature attributed to direct invasion and injury to the disc. Also, studies have shown that puncturing the disc in humans may promote IVD degeneration [ ]. However, CT is faster than MRI but exposure to radiation and consequently poor soft tissue differentiation makes it a choice of investigation only in conditions when MRI is contraindicated.
Magnetic resonance imaging
MRI is considered the gold standard imaging modality for evaluating IVD degeneration and severity (see Chapter 5 ) [ ]. Unlike plain radiographs and CT imaging, MRI does not expose the patient to ionizing radiation yet provides ideal visualization of soft tissue differentiation. Assessment is usually done using both T1- and T2-weighted MRI images. The signal intensity changes due to water contents. A loss of water content reveals a “black disc,” indicative of severe degeneration while a well-hydrated IVD appears bright and plump [ ] on T2. Similarly, other associated changes, such as IVD height loss, displacement and annular tears (formation of high-intensity zone) are also very well recognized with this clinical imaging tool (see Chapters 7–13 ) [ ]. Possibly the most clinically relevant MRI phenotype is Modic change (see Chapter 11 ). These are distinct signal changes due to vertebral endplate alteration. While often accompanied by IVD degeneration, Modic change may contribute independently to LBP [ , ]. Three types of Modic changes help distinguish between acute and chronic phases and have differing clinical implications [ ]. MRI is also able to detect other endplate phenotypes, such as Schmorl’s nodes and fractures [ , ]. In addition, T1-rho or spin lock MRI have also been used for musculoskeletal imaging to facilitate assessing biomarkers for IVD degeneration. These imaging modalities are sensitive to PG profiles and low swelling pressure in the NP [ , ].
The introduction of Fast Low Angle Shot (FLASH) imaging sequences and ultrashort time-to-echo (UTE) imaging has proved valuable examining morphometric features of the CEP [ , ]. Use of FLASH MRI is also suitable for in vivo applications and is able to produce 3D morphology of the CEP [ ]. The morphology of the CEP and defects can be detected using UTE MRI [ ]. The UTE MRI enables visualization and provides complimentary information of those tissues that previously were not visible by MRI [ ], and this advancement allows calcified and noncalcified parts of the CEP to be distinguished [ ]. Dynamic contrast-enhanced (DCE) MRI has also been used for a more quantitative approach of diffusion parameters, ultimately toward the understanding of the disc in health and degeneration [ , ]. Further improvement in the aforementioned diagnostic imaging tools with the passage of time will assist in the early detection of IVD changes.
Classification schemes of disc degeneration
Many in vivo and in vitro grading systems of IVD degeneration have been described in the literature ( Table 6.1 ). The purpose of grading the degenerative changes might be to compare the ability of different evaluating techniques while at the same time correlating the morphological alterations with the functional capability of the IVD [ ]. IVD degeneration has been classified using techniques including radiographs [ , ], discography [ , ], computed tomography [ ], and MRI [ , ]. These imaging modalities are able to categorize the degenerative state of the IVD, largely in the clinical setting with research utility. In vitro studies, by contrast, allow direct macroscopic and microscopic evaluation of the IVD and adjacent structures [ , ].
Classification system | Imaging modality | Grading | Features assessed | Comments |
---|---|---|---|---|
Pfirrmann et al. [ ] | MRI—T2 weighted, sagittal | I–V | Disc structure; distinction of the nucleus and annulus; signal intensity; disc height | Good inter- and intrarater reliability; can be used clinically and in research settings |
Schneiderman et al. [ ] | MRI—T2 weighted, sagittal | 0–III | Signal intensity; disc height; | Commonly used by researchers; correlated with LBP and severity of symptoms |
Lane et al. [ ] | Plain radiographs, lateral view | 0–III | Joint space narrowing; osteophytes; sclerosis | Good inter- and intra-rater reliability. Only requires X-ray radiographs |
Kellgren et al. [ ] | Radiography (cervical) Lateral view | I–IV | Osteophytes; disc narrowing; sclerosis | Validated for classification of cervical disc degeneration |
Well-known macroscopic [ , ] and microscopic assessments [ ] and grading systems allow a more comprehensive analysis but have the least relevance clinically. Owing to the significant contribution of IVD degeneration in LBP, it is pertinent to have a strong grading system based on imaging modalities that can be applicable clinically and have a direct bearing on various pain, disability, and functional phenotypes as well as other outcome measures.
Many plain radiographic methods for grading IVD degeneration have been proposed [ , , ]. These methods have their limitations, especially for disc height and other secondary disc changes [ , ]. Image distortion and angle of lordosis make precise calculations difficult. However, to overcome the problem, various adjustments have been proposed, and the reliability of assessing grades on the basis of IVD height measures, osteophytes, and bony sclerosis in radiographs has been proposed [ , , ].
IVD degeneration can be assessed on T2-weighted MRI, reflecting various grading schemes. One of the more popular grading schemes is that by Pfirrmann et al. ( Table 6.2 , Figs. 6.1 and 6.2 ) [ ]. Based on this scheme, five grades are assigned by looking at the structure and height of the IVD, signal intensity changes, and distinction of NP and AF. This grading system has very good to excellent inter- and intraobserver reliability and is also feasible to use for both clinical and research purposes [ ]. Another scoring system to assess the disc on MRI was reported by Schneiderman et al . [ ], which focuses on the signal intensity of the IVD and notes four grades of degeneration ( Table 6.3 , Fig. 6.2 ). Based on this grading scheme, no signal disc changes correspond to a normal disc while a grade 3 is generally characterized as a “black disc” with narrowed IVD space. This classification is commonly used by researchers and in fact several studies have reported a correlation between LBP and severity of IVD degeneration using this scoring method [ , ].
Grade | Structure | Distinction nucleus and annulus | Signal intensity | Height of intervertebral disc |
---|---|---|---|---|
I | Homogenous, bright white | Clear | Hyperintense, isointense to cerebrospinal fluid | Normal |
II | Inhomogenous with or without horizontal bands | Clear | Hyperintense, isointense to cerebrospinal fluid | Normal |
III | Inhomogenous, gray | Unclear | Intermediate | Normal to slightly decreased |
IV | Inhomogenous, gray to black | Lost | Intermediate to hypointense | Normal to moderately decreased |
V | Inhomogenous, black | Lost | Hypointense | Collapsed disc space |


Grade | Description |
---|---|
Normal–0 | No signal changes |
I | Slight decrease in nucleus pulposus signal intensity |
II | Hypointense nucleus pulposus with normal disc height |
III | Hypointense nucleus pulposus with narrowing disc space |
Although there are numerous classification systems for IVD reported in the literature [ ], the utility of each system depends on several factors, including imaging modality preference and parameters, clinical applicability, as well as the reliability of each system. To clarify which systems were more reliable, Kettler et al. [ ] conducted a systematic review aimed at assessing the inter- and intrarater reliability of each system. They recommended the following imaging classification systems based on their superior inter- and intrarater reliability: Pfirrmann et al. [ ], Schneidermann et al. [ ], Lane et al. [ ], and Kellgren et al. [ ]. These systems are summarized in Table 6.1 .
Recently an Orthopedic Research Society’s Spine Section initiative developed a standardized histopathological classification scheme and a scoring system has been described to establish best practices and methodologies for human disc grading [ ]. This scheme has taken into account all the components of intervertebral discs, including the vertebral endplate, and tried to build a criterion for grading ( Figs. 6.3–6.6 ). In addition, the Spine Section has also reported histopathological disc classification schemes for large animals [ ], rats [ ], and mice [ ].
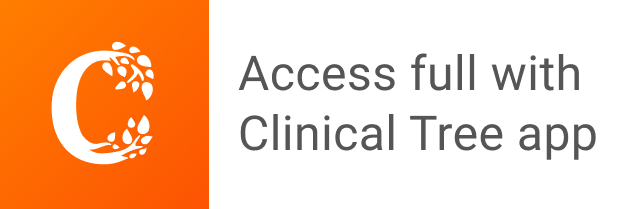