- •
Innate immunity provides the frontline ready-made response to pathogen invasion.
- •
These same pathways are activated in response to other critical insults, including trauma, hypoxic/ischemic injury, and bypass.
- •
Pattern recognition molecules recognize exogenous and endogenous molecular patterns.
- •
Therapeutic interventions in the intensive care unit can have consequences on the innate immune response.
The immune system is a complex, sophisticated defense system that protects the body from danger. Danger may be intrinsic (e.g., cancer) or extrinsic (e.g., pathogenic organisms). The immune system provides constant surveillance to detect potential danger, identifies the nature of the threat, and then mounts a nullifying response. The immune system is also self-regulating—once the threat is nullified, the response needs to be called off.
Traditionally, a distinction has been made between adaptive and innate immune responses. The adaptive immune system has been studied in greater detail and is responsible for a specific response to individual pathogens. Adaptive immunity is a highly sophisticated process with many safety mechanisms to target responses at pathogens (or pathogen-infected cells) while leaving normal tissues alone. This sophistication and specificity take time to develop (days to weeks) and have many effector cells and molecules to deliver a coordinated response, including T and B lymphocytes and immunoglobulins. While the adaptive response is essential for health and in particular for the phenomenon of immunologic memory, it is not sufficient to address a sudden major bacteremia or widespread tissue injury—or even the thousands of minor bacterial inoculums that all individuals experience during their lifetimes (e.g., during teeth brushing). Bacterial growth is exponential under optimal conditions. Neisseria meningitides counts can double in 20 minutes. Given these threats, there is a need for a ready-made system that can act swiftly to neutralize external threats. This is called the innate immune system.
Innate immunity is phylogenetically conserved and found in nearly all multicellular organisms. Again, this contrasts with the adaptive immune system that is found in vertebrates only. In humans, the innate immune system is largely present at birth in contrast to the years it takes to build an adaptive immune repertoire. The hallmarks of the innate immune system are immediacy, promiscuousness, redundancy, and generality. Given that the adaptive immune system evolved in the presence of innate immunity, these systems do not operate in isolation from each other. Rather, the innate immune system presents to and instructs the adaptive part of immunity.
Innate immunity is mediated through immune cells, such as tissue macrophages, neutrophils, and monocytes. It is also mediated through cells that are primarily known for other functions: platelets and endothelial cells. Complex networks of circulating mediators—including the complement cascade, collectins, defensins, and the coagulation cascade—are integral parts of innate immunity. Recent work has highlighted apparent contributions from neurohumoral and autonomic nervous systems.
No critically ill child is admitted to the intensive care unit (ICU) without activation of the child’s innate immune system. No intensivist can function adequately without a good working knowledge of innate immunity, as it is central to many of the clinical entities that the intensivist faces on a day-to-day basis. Infection, trauma, ischemia-reperfusion, acute respiratory distress syndrome (ARDS), and cardiopulmonary bypass sequelae are all largely mediated by the innate immune system.
This chapter provides a framework and outline of concepts in innate immunity that will help in the understanding of some of the pathophysiologic processes central to pediatric critical care.
Components of innate immune system
An ideal first-line response to danger would be pervasive, prompt, effective, and calibrated. The required steps are recognition of a danger signal, dispatch of messengers to mount a response, mounting an effector response that neutralizes danger, and controlling the response to contain damage.
Innate immune stimulus: Danger hypothesis
The traditional paradigm of the immune system suggests that it is programmed, through evolution and adaptation, to differentiate between self and nonself. In this view, invading pathogens such as bacteria would be recognized as nonself and trigger an immune response, while newly replicating blood cells would not trigger a response. However, this does not explain how animals can host entire microbiomes on their mucosal surfaces without inducing an immune response. Similarly, it does not explain how a developing fetus is protected from the maternal immune system.
The danger hypothesis suggests that the immune system does not simply detect self from nonself but instead detects danger or tissue damage. As the first line of defense, the innate immune system must do this rapidly against an almost infinite number of pathogens or noxious molecules. This response is not learned, and it is conserved across phyla.
How does the immune system differentiate between endogenous and pathogenic molecules? The danger hypothesis suggests that it does not. The innate immune response is triggered by molecular patterns that are associated with pathogenic organisms or tissue damage, or both. These signals, known as danger-associated molecular patterns (DAMPs), are a diverse set of molecules (or parts of molecules or polymeric components) sharing recognizable common features that identify them as pathogenic. For example, nucleic acids or mitochondrial proteins released from their intracellular compartment during tissue damage or invading organisms are typical DAMPs. Similarly, hydrophobic protein moieties, or hyppos , are also often hidden within tertiary and quaternary protein structures. When they are exposed, they become DAMPs and trigger an innate immune response.
When associated with pathogens, these molecular patterns signaling danger are known as pathogen-associated molecular proteins (PAMPs). Lipopolysaccharide is an example of PAMPs within the bacterial cell wall, where the hydrophobic part of lipopolysaccharide is hidden. When released by pathogens, this becomes exposed and acts as a PAMP. All hyppos can be DAMPs, including lipid particles and nucleic acids. Protein misfolding, damage, or binding to other molecules (e.g., lipopolysaccharide-binding protein [LBP] binding to lipopolysaccharide) can lead to hyppos being exposed, and normally functional endogenous proteins may become DAMPs. That not all hyppos are always immunostimulatory may be down to the level of aggregation or quantity (evolved from quorum sensing used by eukaryotic colonies such as bacteria—a certain concentration of exposed hyppos will alert the colony of impending danger).
DAMPs released following cell or tissue damage trigger responses from the innate immune system, which are similar to those produced by PAMPs. These endogenous signals are known as alarmins . High-mobility group protein B1 (HMGB-1) is one of the best described alarmins. In an intact cell, HMGB-1 is a histone-associated chromatin protein involved in DNA structural modulation, thereby regulating transcription. However, following necrosis, the intranuclear HMGB-1 is released and recognized by receptors for advanced glycated end products (RAGE), which initiates an immune response aimed to contain damage. Cells have also evolved to release HGMB-1 in response to other signs of danger, which, in the case of ischemia/reperfusion, may lead to actively amplifying the immune response.
Signal recognition
Pattern recognition receptors (PRRs), a group of molecules with repeating sugars or specific amino acid motifs, recognize the typical molecular patterns of DAMPs. PRRs can be soluble circulating molecules, bound to cell surfaces, or intracellular. There is crossover between the types of PRRs: some PRRs, such as CD14, can operate both as a cell surface–bound receptor and in soluble form.
Circulating PRRs include sugar-recognizing collectins, ficolins, and small peptides called antimicrobial peptides . Mannose-binding lectin (MBL) is produced in the liver and activates the complement system after binding typical repeated sugar patterns on pathogen cell walls. The association between MBL levels and the risk of critical illness has not been consistently demonstrated—while some authors describe an increase in risk of the systemic inflammatory response with low levels of MBL, others have not. , Indeed, in some diseases characterized by immune-mediated damage such as mycobacterial infection, moderately low levels of MBL are associated with better protection.
Bacterial permeability increasing protein (BPI) is an antimicrobial protein. It acts against Gram-negative bacteria by increasing cell wall permeability and has been investigated as a therapeutic agent in meningococcal sepsis in children. Although there was no mortality benefit, there was a tendency toward a reduction in sequelae.
Toll-like receptors (TLRs) are the best characterized group of membrane-bound PRRs. There are at least 10 related receptors in humans and 12 in mice ( Fig 100.1 ). TLRs are evolutionarily preserved from the worm Caenorhabditis elegans and strikingly homologous to toll, a gene product essential to Drosophila immunity and dorsoventral patterning. They are characterized by the presence of three distinct domains.

The TLR family can be divided into two subgroups depending on location. TLRs 1, 2, 4, 5, and 6 are bound to cell surfaces and recognize microbial membrane components. The remaining TLRs 3, 7, 8, and 9 are expressed in intracellular membrane-bound organelles and vesicles, such as the endoplasmic reticulum, endosomes, and lysosomes, where they typically recognize nucleic acids from intracellular pathogens.
TLR4 is the best described of the cell surface TLRs given its central role in the pathogenicity of Gram-negative septic shock as a key part of the lipopolysaccharide (LPS) recognition apparatus. In order to bind LPS, TLR4 forms a dimeric complex in association with MD2. This complex formation exposes the binding site that recognizes LPS. LBP binds to LPS, which allows recognition by soluble CD14. CD14 is instrumental in delivering the LPS-bound complex to the TLR4/MD2 complex on the cell surface. TLR4 can also bind to the streptococcal toxin pneumolysin, respiratory syncytial virus (RSV) fusion protein, and paclitaxel, the chemotherapeutic taxol used to treat ovarian, breast, and certain lung cancers ( Fig. 100.2 ).

TLR2 recognizes lipoteichoic acid from gram-positive bacteria, lipoarabinomannan from mycobacteria, zymosan from fungi, and hemagglutinin from measles viruses, among others. TLR2 forms complexes with TLR1 and TLR6 to mediate DAMP recognition. TLR5 is present mainly in gut mucosal dendritic cells and recognizes bacterial flagellin. In doing so, it can activate the adaptive immune system to mount a more specific response to pathogenic bacteria in the gut.
The intracellular membrane-bound TLRs , are instrumental in defending against invasive pathogens. They recognize nucleic acids, especially pathogen-associated nucleic acids such as double-stranded ribonucleic acid (dsRNA) and unmethylated CpG deoxyribonucleic acid (DNA) motifs not seen in mammalian cells. TLR3 recognizes dsRNA during the replication of single-stranded RNA viruses, including RSV. TLR3 deficiency in humans is associated with a susceptibility to infection with herpes simplex virus type 1. TLR7 recognizes RNA viruses, as they are transported in autophagosomes, as well as bacterial RNA from group B Streptococcus. TLR8 is also active against bacteria, and expression can be upregulated in bacterial infection. TLR9 senses bacterial DNA (unmethylated CpG motifs) but also recognizes hemozoin, which is generated after the digestion of hemoglobin by Plasmodium falciparum ( Fig. 100.3 ).

Three major classes of cytoplasmic PRRs are not membrane bound: nucleotide-binding and oligomerization domain-like receptors (NLRs), RIG-I–like receptors (RLR), and absent in melanoma 2–like receptors. NLRs are the best described cytoplasmic PRRs. Similar to TLRs, they have three distinct domains: Knockout mice deficient in one domain of NLRs (NOD-1 −/− mice) are susceptible to Staphylococcus aureus and Helicobacter pylori infections; NOD-2 – deficient mice are prone to infections from Toxoplasma gondii .
Signal transduction
Once PRRs recognize a signal, they need to trigger a response. In order to do so, they send signals to effector pathways using second messengers.
Circulating PRRs can directly activate the complement system via the lectin pathway. MBLs form complexes with MBL-associated serine proteases, which cleave C4 and C2 to form a C3 convertase. C3 convertase cleaves C3 into active components C3a and C3b. This leads to an antiinflammatory cascade, opsonization of phagocytic cells, and the formation of the complement membrane attach complex. Other soluble-protein PRRs such as defensins can act directly as opsonins and chemotactic particles.
Cell surface and intracellular PRRs have Toll/interleukin-1 receptor (TIR)-containing domains that interact with intracellular signaling molecules. Following TLR recognition of DAMPs, the cytosolic TIR-containing domains undergo structural reorganization, which provides a platform for the interaction with a family of adaptor molecules. These include myeloid differentiation primary response 88 (MyD88), the prototypic adaptor molecule involved in the LPS-TLR4 pathway. When bound to activated TLR4, MyD88 recruits a family of kinases, interleukin-1R (IL-1R)–associated kinases (IRAKs), in a complex. Following a cascade of interactions, nuclear factor κB (NF-κB) is activated. The result is the transcription of cytokines leading to an inflammatory response ( Figs. 100.2 and 100.3 ).
As with so many other arms of the innate immune system, there is significant redundancy among adaptor molecules and the signaling pathway. However, MyD88-deficient mice show reduction of NF-κB activation in all TLR pathways apart from TLR3 and TLR4, underlining its importance. The adaptor proteins also have a regulatory function: The adaptor protein sterile-α- and armadillo motif–containing protein (SARM) negatively interacts with the adaptor protein TRIF, limiting the downstream activation of NF-κB.
Effector pathways
For the innate immune system to be effective, it must respond to danger rapidly. The aim of the response is to neutralize the threat where possible, raise the alarm, and seek reinforcements. The effector response can be broadly divided into two arms: the soluble mediator response , which has both widespread systemic effects and more targeted local effects, and the cellular response , which aims to neutralize directly danger through phagocytosis and self-destruction.
Soluble mediator response
The signal transduction pathways described activate nuclear transcription factors such as NF-κB. Cytokine production is an end point of activation of NF-κB. The inflammatory cytokines include tumor necrosis factor–α (TNF-α), IL-1b, IL-4, Il-6, IL-10, IL-12, IL-18, CCL4-RANTES, and transforming growth factor-β. The specific subset of cytokines that is produced and released depends on the innate immune cell type involved. Although NF-κB is the prototypic transcription factor downstream of the innate immune signaling pathways, more recently, transcription factor EB (TFEB) has been described as a potentially important mammalian factor in innate immunity. Unlike NF-κB, TFEB exists in the cytoplasm of macrophages. Upon activation, TFEB moves to the nucleus and leads to transcription of proinflammatory genes. TFEB is increasingly being recognized as a key mediator in both phagocytosis and autophagy (self-destruction). Therefore, TFEB mediates macrophage responses to mycobacteria and bacteria such as S. aureus but also is vital in regulating the response to cellular stress seen in critical illness states.
The soluble mediator arm has a widespread systemic effect as well as a local effect to contain and neutralize the danger signal.
- 1.
Systemic cytokine effects: Systemic effects rapidly follow the exposure to danger. These include the production of fever: TNF-α and IL-1B, among other cytokines, act on the hypothalamus to increase the temperature set point. The fever response is likely to have a beneficial effect in the immune response. In several species, controlling this fever response has a detrimental effect on survival. Observational evidence in humans suggests that the lack of a fever response in critical illness is associated with an increase in mortality. The exact mechanisms of how fever lends itself to immunity are not yet fully elucidated, but it is likely to be multimodal: from decreased pathogenicity of microorganisms to improved immune function. The effects of fever on the immune system include (a) the increased release of neutrophils from the bone marrow; (b) improved localization of neutrophils to tissues; (c) improved phagocytic and cytotoxic activities of neutrophils, macrophages, and natural killer (NK) cells; and (d) increased antigen presentation to T cells by dendritic cells within lymph nodes.
Cytokines also have effects on the circulatory system and nervous system through providing nociceptive signals. This mobilizes energy sources to vital organs to fight the danger and may restrict spread between organisms, respectively. Nervous system involvement may also change behavior to avoid noxious stimuli; for example, TNF-α knockout mice have a decreased perception of bitter taste, implying that the TNF-α sensitizes animals to bitter substances, which may be deemed as noxious.
- 2.
Local neutralization: The complement system, as described earlier, plays an important role in the effector arm of the innate immune system. PRRs activate complement through the lectin pathway. This directly attacks pathogens via the membrane attack complex but also via opsonization and chemokinesis.
Cytokines also have an important role in attracting immune cells to the site of danger; for example, CCL4-RANTES attracts macrophages and NK cells to the site of danger to neutralize the threat. This can lead to a positive feed-forward loop: Cytokines released from local tissue, such as endothelial cells, initially attract immune cells, such as macrophages, to the site of danger. The immune cells then produce more cytokines to amplify the signal and get further reinforcements.
Cellular response
The cellular response involves two processes—phagocytosis and self-destruction. Both aim to neutralize the threat of danger where possible and recruit other immune mechanisms, such as the adaptive immune system.
- 1.
Phagocytosis: DAMP recognition by cell surface PRRs sets into motion cytoskeletal rearrangements. These are achieved by adaptor proteins activating cascades of kinases and guanosine triphosphatases (GTPases), leading to actin polymerization. This results in a phagosome forming and pinching off the cell surface. Activated macrophages are able to internalize >100% of their surface area within 30 minutes. Once internalized, the phagosome may fuse with lysosomes to form a phagolysosome. Here, the DAMPs may be neutralized or processed and relocated to the surface, where they can be presented to T cells to promote an adaptive immune response. Reactive oxygen species (ROS) and nitric oxide are both involved in the neutralization of DAMPS within the phagolysosome. Both are produced during an innate immune response.
- 2.
Self-destruction: Because phagocytosis is the process by which cells internalize and neutralize extracellular material, autophagy is the analogous process for cytoplasmic protein and organelles: the controlled destruction of cellular material following damage. Autophagy is an essential part of cell maintenance and survival: multiorgan failure seen in an inflammatory response is postulated as not being sequelae of disease but rather an adaptive mechanism in response to a reduction in available energy. Autophagy is initiated following damage-induced expression of a family of autophagy-related genes (ATGs). The ATG-encoded proteins assemble to form an autophagosome, a double-membrane structure that envelopes the damaged proteins or organelles. The autophagosome eventually fuses with a lysosome, where the protein or organelle gets enzymatically degraded.
Autophagy may result in cell death once all of the organelles undergo autophagy and the autophagic cell eventually undergoes phagocytosis. This is particularly important following damage, or infection with intracellular pathogens, when the pathogen cannot be controlled. Traditionally, cell death has been described as apoptosis (regulated cell death) and necrosis (accidental cell death). However, as the mechanisms behind cell death have been discovered, this distinction has proved to be an oversimplification. There are currently 10 nonapoptotic described pathways that result in regulated cell death! Some of these occur during normal physiologic processes of development, while others may be triggered by very specific stimuli, such as toxins. It is important to note that regulated cell death can be classified according to whether it is immunogenic (leads to an immune response) or tolerogenic (inhibits an immune response). This is closely related to the release of DAMPs. Many chemotherapeutic agents work by initiating an immunogenic cell death—releasing DAMPs that are then presented to cytotoxic T cells to harness the immune system to kill tumor cells. There is an added degree of control imposed by the post-release modification of DAMPs that can switch an immunogenic cell death to a tolerogenic one. HMGB-1, described earlier, provides a classic example: extracellular HMGB-1 initiates and amplifies an immune response as a DAMP. Once oxidized, HMGB-1 becomes tolerogenic, limiting the anticancer immunogenic response. Similarly, the abundance of HMGB-1, controlled through cleavage and degradation, can also determine the type of immune response it generates.
Crosstalk between systems
The innate and adaptive immune systems interact continuously. Considering them as separate systems is an enormous simplification. Coagulation, neuroendocrine, cardiovascular, and autonomic nervous systems all influence, and are influenced by, immune responses. At the simplest level, this is shown by many molecules having important properties in multiple systems (e.g., acetylcholine is a neurotransmitter and a paracrine regulator of lymphocytes, epinephrine has profound cardiovascular effects but also stimulates the bone marrow to release neutrophils into the circulation). High plasma glucose levels arising from the stress response and insulin resistance during critical illness may inhibit complement binding to and killing of microorganisms.
The interaction between the innate immune system and coagulation cascade is particularly important in critical illness, especially in the context of sepsis and trauma. Plasminogen activator inhibitor 1 (PAI-1) is a potent inhibitor of fibrinolysis. It achieves this response by inhibiting both tissue- and urinary-type plasminogen activator. Levels of PAI-1 are increased after trauma and sepsis, especially so in severe meningococcal sepsis. Inflammatory mediators TNF-α, IL-1 and IL-6, complement 5a, and LPS all act to increase PAI-1 production. In turn, PAI-1 contributes to a procoagulant state and inhibits neutrophil apoptosis. Although this may help to contain inflammation at the site of infection, genotypes associated with high levels of PAI-1 production are associated with worse outcome in septic shock. Thus, there is a direct link between how readily the immune system triggers an increased clotting tendency in critical illness and poor outcome.
Similarly, inflammatory mediators such as IL-6 and HMGB-1 stimulate release of the potent coagulation activator tissue factor (TF) from activated monocytes, macrophages, and endothelial cells. Small membrane vesicles from apoptotic cells known as microparticles bind to cell surfaces through specific receptors, expressing TF. This promotes thrombin formation, which, in turn, converts fibrinogen to fibrin. Thrombin and fibrin generation are increased in inflammation, in part because fibrinolysis is impaired due to increased activity of PAI-1 but also secondary to diminished activated protein C (APC) and tissue factor pathway inhibitor (TFPI). These processes have been the targets for numerous clinical trials of drugs with anticoagulant/profibrinolytic actions—all aiming to achieve antiinflammatory effects by targeting coagulation systems. APC may also have other antiinflammatory actions, downregulating inflammatory cytokines, preventing the loss of the endothelial barrier, and acting as an antioxidant and antiapoptotic agent. Although initial trials suggested therapeutic benefits of APC in sepsis, this has since been contradicted in larger trials in both children and adults.
Another example of a novel interaction between the coagulation and immune systems is that TLR4-activated platelets interact with neutrophils to trap and kill bacteria in so-called neutrophil extracellular traps ( Fig. 100.4 ) . In vitro studies showed that LPS, as well as plasma from septic adults, could induce this phenomenon.
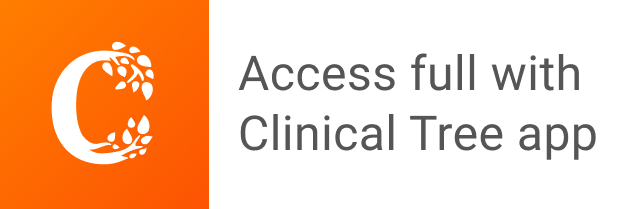