Innate immunity depends on recognition of conserved molecular patterns found in many microorganisms.
Several families of pattern recognition receptors are responsible for triggering innate immune responses.
Toll-like receptors and other pattern recognition receptors with leucine-rich repeat domains play a key role in innate immune recognition.
Anti-microbial peptides are important effectors of innate immunity.
Phagocytic cells and several types of innate-like lymphocytes are key cell types in mediating innate immunity.
Innate immune responses have a strong impact on the development of adaptive immunity.
Some defects in the innate immune system are associated with a predisposition to infection or to autoimmune disease.
Introduction
It has become common practice in immunology to divide the mechanisms involved in host defense into adaptive and innate components. This approach provides a useful framework for classifying the numerous cells, receptors, and effector molecules that combine to make up the vertebrate immune system ( Table 20.1 ). A specific immune response, such as the production of antibodies or T cells against a particular pathogen, is referred to as adaptive immunity because it represents an adaptation that occurs during the lifetime of an individual as a result of exposure to that pathogen. Adaptive immune responses involve the clonal expansion of T and B lymphocytes bearing a large repertoire of somatically generated receptors that can be selected to recognize virtually any pathogen. The adaptive immune system of any given person is profoundly molded by the immunologic challenges encountered by that person during the course of a lifetime. A hallmark of adaptive immune responses is that they are highly specific for the triggering agent, and they provide the basis for immunologic memory. This property of memory endows the adaptive immune response with its “anticipatory” property, which provides increased resistance against future infection with the same pathogen and also allows vaccination against future infectious threats.
Property | Innate Immune System | Adaptive Immune System |
---|---|---|
Receptors | Relatively few (several hundred?) | Many (potentially 10 14 or more) |
Fixed in genome | Encoded in gene segments | |
Gene rearrangement not required | Gene rearrangement required | |
Distribution | Nonclonal | Clonal |
All cells of a class identical | All cells of a class distinct | |
Targets | Conserved molecular patterns | Details of molecular structure |
Lipopolysaccharides | Proteins | |
Lipoteichoic acids | Peptides | |
Glycans and peptidoglycans | Carbohydrates | |
Others | ||
Self–non–self-discrimination | Perfect: selected over evolutionary time | Imperfect: selected in individual somatic cells |
Action time | Immediate or rapid (seconds to hours) | Delayed (days to weeks) |
Response | Microbicidal effector molecules | Clonal expansion or anergy of specific T and B lymphocytes |
Anti-microbial peptides | Cytokines (IL-2, IL-4, IFN-γ, others) | |
Superoxide | Specific antibody production | |
Nitric oxide | Specific cytolytic T cell generation | |
Cytokines (IL-1, IL-6, others) | ||
Chemokines (IL-8, others) |
Adaptive immunity is essential for the survival of all mammals and most other vertebrates, but a wide variety of other mechanisms that do not involve antigen-specific lymphocyte responses are also involved in successful immune protection. These diverse mechanisms are collectively known as innate immunity because they are not dependent on prior exposure to specific pathogens for their amplification. Such responses are controlled by the products of germline genes that are inherited and similarly expressed by all healthy people. Innate immune mechanisms involve both constitutive and inducible components and use a wide variety of recognition and effector mechanisms. It has become clear in recent years that innate immune responses have a profound influence on the generation and outcome of adaptive immune responses. This ability of the innate immune system to instruct the responses of the adaptive immune system suggests many ways in which innate immunity can influence the development of both long-term specific immunity and autoimmune disease.
Evolutionary Origins of Innate Immunity
In spite of its obvious importance for most vertebrate organisms, the adaptive immune system is a relatively recent evolutionary development ( Fig. 20.1 ). In the great majority of present-day vertebrate species, the adaptive immune system is based on the ability to generate large families of variable lymphocyte receptors with immunoglobulin-like structures. This ability has been conserved as a result of the acquisition of a specialized recombination system that mediates the assembly of gene segments in the T cell and B cell receptor families, which most likely occurred through invasion of the genome of a primitive vertebrate by a transposable element or virus carrying this machinery. This critical step in the evolution of the immune system can be traced back to the emergence of the ancestors of present-day jawed fish, which represent the most primitive extant species known to have adaptive immune systems based on the generation of large families of specific immunoglobulin-type receptors. Recently, other systems of variable lymphocyte receptors that are unrelated to immunoglobulins but also provide the basis for an adaptive immune response have been discovered in primitive jawless fish such as lampreys and hagfish. This finding shows that at least two different strategies for the creation of an adaptive immune system emerged at the dawn of vertebrate evolution about 500 million years ago, and it emphasizes the importance of adaptive immunity for survival and further evolution of the vertebrate lineages.

Given this key role of adaptive immunity in the evolution and survival of vertebrates, it is surprising that all invertebrate animals appear to lack the ability to generate lymphocyte populations bearing large families of clonally diverse antigen receptors. In these animals, protection against pathogen invasion depends entirely on innate immunity, elements of which appear to exist in all animals and plants and must have evolved with the earliest multicellular forms of life. In many cases, components of the innate immune system are significantly conserved in structure and function in animals from the lowliest invertebrates to the most complex vertebrates. This preservation of innate immune mechanisms, with their functions largely intact, over such vast evolutionary distances is a clear indication of their importance, even in animals that have developed sophisticated adaptive immune responses.
Pathogen Recognition by the Innate Immune System
Some mechanisms of innate immunity are constitutive, meaning that they are continuously expressed and are not significantly modulated by the presence or absence of infection. Examples include the barrier functions provided by epithelial surfaces continuously exposed to microbial flora, such as those of the skin and intestinal and genital tracts. In contrast, the inducible mechanisms of innate immunity involve increased production of mediators and upregulation of effector functions that eliminate microorganisms. Induction occurs as a result of exposure to a wide variety of microbes and represents a less specific form of immune recognition than that associated with the specific antibodies and T cells that mediate adaptive immunity. The basic principle underlying this form of response is a process known as pattern recognition. This recognition strategy is based on the detection of commonly occurring and conserved molecular patterns that are essential products or structural components of microbes.
PAMPs and DAMPs: Patterns for Innate Immune Recognition
Pathogen-Associated Molecular Patterns
The general name given to the targets of innate immune recognition in microbes is pathogen-associated molecular patterns (PAMPs). These structural features or components distinctive for microorganisms are not normally found in the animal host. The best-known example of a PAMP is bacterial lipopolysaccharide (LPS), a ubiquitous glycolipid constituent of the outer membranes of gram-negative bacteria. Another important example is the peptidoglycan structure present as the basic cell wall component in nearly all bacteria. These structures may vary partially from one bacterium to another, but the basic elements are conserved, thus providing the possibility of recognizing a broad array of pathogens by sensing a single or a relatively small number of PAMPs. Many PAMPs that serve as targets of recognition for the innate immune response are now known to be associated with bacteria, fungi, and viruses.
In addition to allowing direct recognition of molecules produced by various microorganisms, the innate immune system is able to respond to the patterns of host-derived molecules released by cells undergoing necrotic death. The molecules recognized are generally referred to as damage-associated or danger-associated molecular patterns (DAMPs) and include multiple different families of proteins, as well as nonproteinaceous substances such as uric acid microcrystals. Thus the response to DAMPs can be an indirect response to microbial invasion, or it can be triggered by other types of tissue damage such as ischemia to result in sterile inflammation.
Pattern Recognition Receptors
Recognition of PAMPs and DAMPs is mediated by a collection of germline-encoded molecules known collectively as pattern recognition receptors (PRRs) ( Table 20.2 ). These receptors are host proteins that have evolved, through many millions of years of natural selection, to possess precisely defined specificities for particular PAMPs or DAMPs expressed by microorganisms. The total number of PRRs present in complex vertebrates such as humans is estimated to be several hundred—a number limited by the size of the genome of any animal and the number of genes it can dedicate to immune protection. The human genome, for example, is estimated to contain approximately 19,000 to 20,000 protein-coding genes, most of which are not related directly to the immune system. This example demonstrates one of the strong points of contrast between the innate and adaptive immune systems, because the latter can possess in the range of 10 14 different somatically generated receptors for foreign antigens in the form of antibodies and T cell receptors. With its much more limited array of receptors, the innate immune system uses the strategy of targeting highly conserved PAMPs that are shared broadly by large classes of microorganisms. Because most pathogens contain PAMPs, this strategy allows the generation of at least partial immunity against most infections.
Receptor Class | Examples | Prominent Sites of Expression | Major Ligands | Function |
---|---|---|---|---|
Secreted PRRs | Collectins Mannan-binding lectin Ficolins Surfactant proteins (SP-A, SP-B) Pentraxins Short pentraxins (CRP, SAP) Long pentraxins | Plasma | Carbohydrate arrays typical of bacterial capsules, fungi, and other microbes Apoptotic cells and cellular debris, including chromatin | Complement activation Opsonization |
Endocytic PRRs | Lectin-family receptors Macrophage mannose receptor DEC-205 Dectin-1 Scavenger receptor A MARCO Complement receptors CD11b/CD18 (CR3) CD21/35 (CR2/1) | Macrophages, dendritic cells, some endothelia, epithelia, and smooth muscle cells | Cell wall polysaccharides (mannans and glucans), LPS, LTA, and opsonized cells and particles | Pathogen uptake by phagocytes Delivery of ligands to antigen-processing compartments Clearance of cellular and extra-cellular debris |
Signaling PRRs | Toll-like receptors Nod-like receptors Pyrin domain proteins PYHIN proteins RIG-I-like receptors | Macrophages, dendritic cells, epithelia | Multiple conserved pathogen-associated molecular patterns (LPS, LTA, dsRNA, lipoproteins, flagellin, viral or bacterial DNA, others) | Activation of inducible innate immunity (anti-microbial peptides, cytokines, reactive oxygen or nitrogen intermediates) Instruction of adaptive immune response |
PRRs are expressed by many cell types; some are specialized effector cells of the immune system (e.g., neutrophils, macrophages, dendritic cells, and lymphocytes), and others are not generally regarded as part of the immune system (e.g., epithelial and endothelial cells). Unlike the T and B cell receptors used for adaptive immune recognition, expression of PRRs is not clonal, which means that all receptors displayed by a given cell type (e.g., macrophages) have identical structure and specificity. When PRRs are engaged by recognition of their associated PAMPs or DAMPs, effector cells bearing the PRRs are triggered to perform their immune effector functions immediately, rather than after undergoing proliferation and expansion, as in the case of adaptive immune responses. This mechanism accounts for the much more rapid onset of innate immune responses.
Considerable progress has been made toward identifying many of the important PRRs involved in the induction of innate immunity. These receptors can be classified into three functional classes: secreted, endocytic, and signaling PRRs (see Table 20.2 ). In addition, many of the known PRRs can be classified into structurally defined families on the basis of a few characteristic protein domains. Among these, the best known include proteins with calcium-dependent lectin domains, scavenger receptor domains, and leucine-rich repeat domains (LRRs).
Pattern Recognition Receptors of the Lectin Family
Calcium-dependent lectin domains are common modules of secreted and membrane-bound proteins involved in the binding of carbohydrate structures. A well-characterized PRR belonging to this class is the mannan-binding lectin (MBL), also known as soluble mannose-binding protein, which represents a secreted PRR that functions in initiation of the complement cascade ( Fig. 20.2 ). This protein is synthesized primarily in the liver on a constitutive basis, although its production can be increased as an acute phase reactant after many types of infection. MBL binds to carbohydrates on the outer membranes and capsules of many bacteria, as well as fungi, some viruses, and parasites. Although mannose and fructose sugars bound by MBL can also be found on the surfaces of normal mammalian cells, they are present at too low a density or in the wrong orientation to efficiently engage the lectin domains of MBL. In contrast, the coats of many microorganisms contain an array of these sugars, which allows strong binding of MBL. Thus, in this case, the spacing and orientation of specific carbohydrate residues constitute the PAMP that triggers the activation of innate immunity by MBL. MBL functions as one of a small number of secreted PRRs that can initiate the lectin pathway of complement activation. At least two other soluble proteins with lectin activity in human plasma, known as ficolins (ficolin/P35 and H-ficolin), can also activate this pathway after their interaction with bacterial polysaccharides.

Several of the soluble lectin-type PRRs also play an important role in the opsonization of microbes by binding to their surfaces and directing them to receptors on phagocytic cells. Among these are two pulmonary surfactant proteins, SP-A and SP-D, which similarly recognize and bind to the surface sugar codes of microbes in the respiratory tract. These molecules are similar in structure to MBL, having both collagen-like and lectin domains, and together they constitute a family of soluble PRRs known as collectins. Another family of soluble PRRs that performs a similar function in plasma is the pentraxins, so called because they are formed by the association of five identical protein subunits. This family includes the acute-phase reactants C-reactive protein (CRP) and serum amyloid P protein (SAP), along with a number of so-called long pentraxins , which have an extended polypeptide structure with homology to the classic short pentraxins (i.e., CRP and SAP) only at their carboxy-terminal domains. Long pentraxins are expressed in a variety of different tissues and cells, and their specific functions are mostly unknown. However, the long pentraxin PTX3 plays an important, nonredundant role in resistance to fungal infection in mice, and recent studies indicate that PTX3 is essentially a functional ancestor of antibodies that recognizes microbes and promotes their clearance through complement activation and phagocytosis.
In addition to these soluble proteins, a large number of membrane-bound glycoproteins with lectin domains are known to exist; some of them participate in innate immunity by serving as endocytic PRRs for the uptake of microbes or microbial products (see Fig. 20.2 ). One of the most extensively studied of these is the macrophage mannose receptor (MMR). Although originally identified on alveolar macrophages and known to be expressed on macrophage subsets throughout the body, this receptor is also expressed on a variety of other cell types, including certain endothelia, epithelia, and smooth muscle cells. The MMR is a membrane-anchored, multilectin domain–containing protein that mediates the binding of a broad range of pathogens, leading to their internalization via endocytosis and phagocytosis. Although the major function of the MMR appears to be directing the uptake of its ligands, evidence suggests that this receptor may be capable of signaling to modify macrophage functions after receptor engagement. Another member of this receptor family, the β-glucan binding cell-surface lectin known as dectin-1, has a role in the modulation of inflammation in a mouse model of infection-induced arthritis.
Pattern Recognition Receptors of the Scavenger Receptor Family
The scavenger receptor family contains a broad range of structurally diverse cell surface proteins that are expressed most prominently on macrophages, dendritic cells, and endothelial cells (see Fig. 20.2 ). Although they were originally defined by their ability to bind and take up modified serum lipoproteins, they also bind a wide range of other ligands, including bacteria and some of their associated products. Multiple members of this family have been implicated as PRRs for innate immunity, including the scavenger receptor A (SR-A) and a related molecule called the macrophage receptor with collagenous structure (MARCO). Both of these molecules contain a scavenger receptor cysteine-rich domain in the distal ends of their membranes and a collagen-like stalk with a triple-helical structure. Both are known to bind bacteria, and SR-A also binds well-known PAMPs such as lipoteichoic acids and LPS. Mice that have been made deficient in SR-A by targeted gene disruption show increased susceptibility to infections caused by a variety of bacteria, thus providing strong evidence of the role of scavenger receptors in protective immunity, most likely through the activation of innate immune mechanisms. Members of the class B scavenger receptor family, including CD36 and SR-BI/CLA-1, have also been found to recognize a variety of pathogen-derived molecules. Although these members of the scavenger receptor family clearly function as endocytic PRRs in the uptake of microbes, their potential to serve as signaling receptors has not yet been established. However, several scavenger receptors play a co-receptor role in the signal transduction process mediated by members of the Toll-like receptor (TLR) family (discussed in a later section), most likely by capturing specific ligands and transferring them to adjacent TLRs.
Pattern Recognition Receptors With Leucine-rich Repeat Domains
LRRs are structural modules found in many proteins, including PRRs involved in signaling the activation of innate immunity. Molecules in this class include, most notably, the family of mammalian TLRs, which are membrane-bound signal-transducing molecules that play a central role in the recognition of extra-cellular and vacuolar pathogens. Two families of cytoplasmic LRR-containing receptors have also been identified; they play a prominent role in the innate immune recognition of PAMPs expressed by intra-cellular pathogens. These include the families of caspase activation and recruitment domain (CARD) proteins and of pyrin domain proteins. These molecules are closely related in structure and function to proteins found in invertebrates and plants involved in pathogen resistance, highlighting the ancient origin of these pathways for host defense; they appear to have been recognizably conserved throughout approximately 1 billion years of evolution.
Toll-like Receptors
The first member of the Toll family to be discovered was the Drosophila Toll protein, which was identified as a component of a signaling pathway that controls dorsoventral polarity during development of the fly embryo. The sequence of Toll showed it to be a transmembrane protein with a large extra-cellular domain containing multiple tandemly repeated LRRs at the N-terminal end, followed by a cysteine-rich domain and an intra-cellular signaling domain ( Fig. 20.3 ). A role for Toll in immune responses was suggested by the observation that its intra-cellular domain shows homology to the mammalian IL-1R cytoplasmic domain. This association was later confirmed in studies showing that Toll was critical for the antifungal response in the fly, linking this pathway for the first time to innate immunity. Identification of Drosophila Toll eventually led to a search for similar proteins in mammals; this effort has been richly rewarded, yielding a family of 10 TLRs in humans and 12 in mice. Among these, TLR1 through TLR9 are conserved between mice and humans, TLR10 is present only in humans, and TLR11 through TLR13 are expressed only in mice. All these molecules contain large extra-cellular domains with multiple LRRs, as well as intra-cellular signaling domains known as Toll/IL-1R, or TIR, domains. Many of these TLRs have been linked to innate immune responses against various PAMPs of different microorganisms.

Toll-like Receptor 4 and the Response to Lipopolysaccharide
The first human TLR to be identified was the molecule now designated TLR4, which is a major component in the response to one of the most common of all PAMPs: bacterial LPS. Earlier studies on the response to LPS had identified two proteins, CD14 and LPS-binding protein, as molecules involved in the binding of LPS to the surface of LPS-responsive cells. However, these molecules did not possess any potential for transducing signals into the cell, so it was unclear how LPS binding would lead to the activation of cellular responses associated with gram-negative bacterial infection. The answer was provided by positional cloning studies of the gene responsible for the phenotype in the LPS-hyporesponsive C3H/HeJ mouse. This study revealed a single amino acid substitution in the signaling domain of TLR4. Specific deletion of the TLR4 gene by targeted gene disruption in mice subsequently confirmed the essential role of this molecule in the response to LPS, because TLR4 knockout mice have almost no response to LPS and are highly resistant to endotoxic shock. Biochemical studies provide further support for TLR4 as a component of the LPS receptor; they show that LPS bound to the surface of cells is in close contact with both CD14 and TLR4, as well as another protein called MD-2, which appears to perform an accessory function in the binding of LPS to the receptor complex. Additional studies have elucidated many of the downstream elements in the signaling pathways that connect TLR4 to the activation of genes associated with inducible innate immunity. Studies of Toll signaling pathways in Drosophila have identified the transcription factor nuclear factor-κB (NF-κB) as one of the key effectors of gene activation after the engagement of Toll. This basic pathway in the fly appears to be largely conserved in TLR signaling in higher animals, including mammals.
Other Pathogen-Associated Molecular Patterns Recognized by Toll-like Receptors
The search for ligands that lead to signaling through various TLRs has demonstrated that this family of PRRs is collectively responsible for innate immune responses to an extraordinary array of PAMPs. In addition to its central role in the signaling of responses to LPS, TLR4 is involved in responses to multiple different self-ligands and non–self-ligands. The anti-mitotic agent and cancer chemotherapy drug paclitaxel (Taxol) mimics LPS-induced signaling in mouse cells through a pathway that requires both TLR4 and MD-2. Other foreign ligands of TLR4 include the fusion protein (F protein) of respiratory syncytial virus and the heat shock protein 60 (HSP60) of chlamydia. TLR4 can signal in response to mammalian HSP60, a protein expressed at increased levels and most likely released by stressed or damaged cells. This represents a variation of the pattern recognition principle in which the pattern is an endogenous molecule released by damaged host cells that serves as a DAMP, rather than a PAMP produced directly by a pathogen. Other examples of recognition of DAMPs by TLR4 include responses to oligosaccharide breakdown products of tissue hyaluronans and responses to the extra-domain A region of fibronectin produced by alternative RNA splicing in response to tissue injury or inflammation.
The range of PAMPs recognized through TLR2 is probably even greater than for TLR4. TLR2 is known to be involved in signaling in response to multiple PAMPs of gram-negative and gram-positive bacteria, including such structures as bacterial glycolipids, bacterial lipoproteins, parasite-derived glycolipids, and fungal cell wall polysaccharides. TLR2 does not function independently in responding to these PAMPs; rather, it forms heterodimers with TLR1 or TLR6. This ability to pair with other TLRs appears to be unique to TLR2, because other TLRs that have been studied carefully (e.g., TLR4 and TLR5) most likely function only as monomers or homodimers. Other TLRs with currently defined ligands are TLR5 (involved in the response to bacterial flagellin), TLR3 (double-stranded RNA), TLR7 (single-stranded RNA), and TLR9 (unmethylated bacterial DNA). Most, if not all, microbes contain multiple PAMPs that are recognized by different TLRs. For example, a typical bacterium expressing LPS also contains unmethylated DNA and thus generates signals not only through TLR4 but potentially through TLR9 as well. Because different TLRs are capable of activating distinct signaling cascades, the ability of a single cell to detect several different features of a pathogen simultaneously with multiple TLRs may help the innate immune response to be more finely tuned to respond to a particular challenge.
NLR, STING, and RLR Proteins
There are a large number of cytosolic proteins that have structural similarities to membrane-bound TLRs and that function as sensors for PAMPs of intra-cellular pathogens and as regulators of innate immune responses (see Fig. 20.3 ). Many of these proteins contain LRR domains and have been classified on the basis of their incorporation of a CARD or pyrin domain. The nomenclature and classification schemes for this growing family of innate immune sensors and regulators are still evolving, although the most recent literature shows a trend toward referring to this group of proteins as the NLR family, an acronym that stands for either “nucleotide-binding domain, leucine-rich repeat proteins” or “Nod-like receptors.” The first intra-cellular microbial sensors in this family to be described were the Nod1 and Nod2 proteins, which contain LRR domains linked to a central nucleotide binding domain (NBD, also designated as NOD or NACHT domains ) and an N-terminal CARD domain. As in the case of TLRs, the LRR domains of these proteins appear to be involved in the direct recognition of pathogen-derived molecules and a variety of host components that function as DAMPs, and their CARD domains are linked to downstream signaling for the activation of innate immunity. Although originally implicated in the response to bacterial LPS, it is now well accepted that both Nod1 and Nod2 are primarily involved in the recognition of muropeptide monomers released from bacterial cell wall peptidoglycans. Signals resulting from the recognition of peptidoglycan components by Nod1 and Nod2 lead to activation of the NF-κB pathway, as in the case of TLR signaling. However, other signaling pathways also appear to be engaged, such as activation of procaspase-1 and caspase-9 by CARD domain interactions, leading to increased production of IL-1β and cell death through a process referred to as pyroptosis.
The pyrin domain–containing proteins represent a major subgroup of NLRs that are believed to signal in response to microbial invasion or cellular stress. The prototype member of this family is pyrin, which is the product of the gene that is mutated in people with familial Mediterranean fever. Although pyrin itself lacks an LRR domain, numerous other members of this family contain an LRR linked to a central NOD domain and an N-terminal pyrin domain. These family members include cryopyrin (also known as NLRP3 or NALP3 ), which is mutated in patients with a range of hereditary inflammatory diseases referred to collectively as cryopyrin-associated periodic syndromes (CAPS). Cryopyrin, together with these multiple related proteins, constitutes a large set of related proteins known as the NLRP (NLR-PYRIN domain) or NALP (NACHT-LRR-PYRIN domain–containing proteins) family. The human genome contains 14 genes encoding NLRP proteins, the precise functions of which are still largely unknown. However, several NLRP proteins, particularly NLRP3 and NLRP1, and also the closely related NAIP protein, have been identified as key components in the formation of intra-cellular complexes known as inflammasomes. These cytosolic protein complexes serve as activating platforms that are involved in the activation of caspases—that is, intra-cellular proteases required for the processing of inflammatory cytokines such as IL-1β and IL-18. Direct recognition of specific PAMPs by NLRP proteins remains to be established, although initial studies implicate these proteins as direct or indirect sensors of various stimuli, including constituents of bacteria (peptidoglycan, bacterial RNA, and exotoxins), viruses (double-stranded RNA), and uric acid crystals.
In addition to the likely role of NLRP proteins in sensing foreign nucleic acids as PAMPs, recent studies have characterized a complex array of other proteins that serve as sensors for intra-cellular DNA or RNA and are likely to play major roles in anti-viral innate immunity. One prominent example is the family of PYHIN proteins, which are cytosolic or nuclear proteins containing PYRIN domains linked to a DNA-binding domain known as HIN-200. Some of these proteins, such as the absent in melanoma-2 (AIM2) protein, have been clearly established to act as sensors for intra-cellular viral DNA that can trigger a range of innate immune responses. A range of other candidate intra-cellular proteins have been identified that make important contributions to the innate response to DNA, particularly those that act through signaling pathways mediated by the adaptor protein called stimulator of interferon genes (STING). STING is a transmembrane dimeric protein in the endoplasmic reticulum membrane that weakly recognizes double-stranded (ds) DNA but is strongly activated by cyclic dinucleotides, which can either be directly produced by intra-cellular bacteria as PAMPs or generated as DAMPs by the cyclic guanosine monophosphate–adenosine monophosphate (GMP-AMP) synthase cGAS when it is activated by binding cytosolic dsDNA. In addition to these cytosolic DNA sensors, there is a growing list of cytosolic RNA sensing proteins; the most well studied at present are the RIG-I-like receptors (RLRs). The RLRs include the retinoic acid–inducible gene-I (RIG-I), melanoma differentiation factor 5 (MDA5), and laboratory of genetics and physiology 2 (LGP2) proteins. These proteins are expressed in the cytosol of most cells and respond to structural motifs present in many viral RNA molecules to induce type I interferon (IFN) and other anti-viral effectors. Together, these multiple sensors of cytosolic nucleic acids serve to trigger inflammatory responses against a large spectrum of microbial pathogens, including DNA and RNA viruses, and to make potentially important contributions to autoimmune and inflammatory diseases.
Effector Mechanisms of Innate Immune Responses
The ability to recognize pathogens through PRRs allows activation of numerous anti-microbial effector mechanisms by the innate immune response. These responses lead to the killing of pathogens through production of effector molecules with direct microbicidal activities, including the membrane attack complex of complement, a variety of anti-microbial peptides, and the caustic reactive oxygen and reactive nitrogen intermediates generated within phagocytic cells. In invertebrates, these mechanisms represent virtually the entire protective response against microbial invaders. However, in most vertebrates, including mammals, innate immune recognition also has profound effects on triggering and programming the adaptive immune response that follows somewhat later. This ability of the innate immune system to instruct the adaptive response has major implications for the development of long-term protective immunity to infection and may play a critical role in mechanisms leading to autoimmunity.
Cell Types Mediating Innate Immunity
Many types of cells have the ability to mount at least a limited response to PAMPs, but the most effective cell types in this regard are the specialized phagocytes, such as macrophages, neutrophils, and dendritic cells. Upon recognition of microbial stimuli, these cells have the ability to upregulate nicotinamide adenine dinucleotide phosphate (NADPH) oxidase by assembling the components of this enzyme complex on phagosomal membranes, leading to an oxidative burst that produces microbicidal superoxide ions. Many phagocytic cells also increase their expression of inducible nitric oxide synthase (iNOS, or NOS2) upon contact with various PAMPs. This increased expression leads to the production of reactive nitrogen intermediates, including nitric oxide and peroxynitrite, which have potent direct antimicrobicidal activities. These responses are synergistic because the anti-microbial activity of the phagocyte oxidase system is frequently enhanced by the expression of reactive nitrogen intermediates.
Innate-like Lymphocytes
A number of distinct lymphocyte subsets also play important roles in innate immune responses. Among these subsets are a complex mixture of lymphocytes that lack markers of the T and B cell lineages and are collectively referred to as innate lymphoid cells (ILCs). The complexity of ILC populations is currently still being unraveled. Recent classifications recognize three distinct lineages designated ILC1, ILC2, and ILC3 cells that are distinguished by phenotypic markers and by secretion of different cytokines. The cytokine secretion profiles of these ILC subsets and some aspects of their functions in immunity parallel closely those of conventional T helper cell subsets (i.e., Th1, Th2, and Th17 cells). The ILC1 subset is composed of IFN-γ–secreting populations, which include natural killer (NK) cells. These lymphocytes do not express receptors generated by somatic recombination and thus depend on germline-encoded receptors for signaling their responses against pathogen-infected cells. NK cells participate in the early innate response against virally, and probably bacterially, infected cells through expression of cytotoxic activity and secretion of cytokines.
Several other subsets of lymphocytes belonging to the T and B cell lineages have been identified as participants in the rapid response against pathogens to which the host has not previously been exposed. Although these cells express clonally variable, somatically rearranged antigen receptors (T cell antigen receptors or membrane immunoglobulins) and thus could be classified as components of the adaptive immune system, their manner of functioning is much more characteristic of innate than adaptive immunity. These innate-like lymphocytes (ILLs) may represent remnants of the earliest primitive adaptive immune system, and they appear to have been conserved to varying degrees because they continue to make specialized contributions to host immunity.
Among the currently recognized ILLs are two B cell populations, known as the B1 and marginal zone B cell subsets . These B cell populations are involved in the spontaneous production of natural antibodies, which are largely germline-encoded immunoglobulins that are reactive to commonly expressed microbial determinants. In addition, both of these B cell populations generate rapid T cell–independent responses after bacterial challenges and thus contribute to the first line of immune defense that precedes the onset of adaptive immunity. A subset of B1 cells is the Innate Response Activator (IRA) B cells; this unique subset originates from serosal sites and co-secretes substantial amounts of granulocyte-macrophage colony-stimulating growth factor (GM-CSF) and IL-3 upon bacterial LPS recognition in the spleen and the lung. While IRA B cell-derived GM-CSF protects against bacterial sepsis by promoting protective IgM production and increased phagocyte clearance, it can also drive pathologic changes such as atherosclerosis by enhancing splenic extramedullary hematopoiesis and pro-inflammatory phagocyte production.
Among the T cells, three populations of ILLs have been identified and characterized in detail: γδ T cells, NK T cells, and mucosal-associated invariant T (MAIT) cells. The γδ T cells express somatically rearranging receptors that use a limited number of variable region genes and are thought to recognize a narrow spectrum of foreign or self-ligands. In humans, the specificities of two subsets of γδ T cells have been at least partially defined. One of these subsets, the major circulating population expressing the Vδ2 gene product, responds rapidly and without prior immunization to a variety of small alkyl phosphate and alkyl amine compounds that are produced by many bacteria. Another subset, characterized by its expression of the Vδ1 gene product, responds to class I major histocompatibility complex (MHC)–related self-molecules of the class I MHC chain–related A and B (MICA/B) and CD1 families. These molecules may serve as markers of cellular stress and are upregulated on cells in the context of infection or inflammation, leading to the activation of Vδ1-bearing γδ T cells.
A similar principle appears to be involved in the functioning of NK T cells, which are so named because of their co-expression of an αβ T cell antigen receptor and a variety of receptor molecules that are typically associated with NK cells. Similar to γδ T cells, NK T cells have somatically rearranged antigen receptors that use a limited array of V genes and most likely recognize a narrow range of foreign or self-antigens. A major population of NK T cells is reactive with the class I MHC–like CD1d molecule, and these ILLs are activated by recognition of a variety of lipid or glycolipid ligands that can be presented by CD1d. Several bacterial glycolipids have been identified as specific antigens that stimulate NK T cells, suggesting that these cells may be rapid responders that contribute to innate antibacterial immunity. A wide variety of mouse disease models have shown that NK T cells also make significant contributions to the development of adaptive immune responses and may play a particularly important role in immunoregulation to prevent autoimmunity. Similarly, MAIT cells are a separate population of innate-like T cells that have recently been shown to use their αβ T cell antigen receptors to recognize common vitamin B metabolites that are produced and secreted by a wide range of bacteria.
Anti-microbial Peptides
Anti-microbial peptides are the key effector molecules of inducible innate immunity in many invertebrates and are being increasingly recognized as important elements of innate immunity in higher animal species, including mammals. They are evolutionarily ancient components of host defense that are widely distributed throughout all multicellular organisms in the animal and plant kingdoms. More than 1000 such peptides have been identified, and their diversity is so great that it is difficult to categorize them. However, at a structural and mechanistic level, most of these peptides share several basic features. They generally are composed of amino acids arranged to create an amphipathic structure with hydrophobic and cationic regions. The cationic regions target a fundamental difference in membrane design between microbes and multicellular animals, which is the abundance of negatively charged phospholipid head groups on the outer leaflet of the lipid bilayer. The preferential association of anti-microbial peptides with microbial membranes leads to membrane-disrupting activity, most likely involving the interaction of the hydrophobic regions of the peptide with membrane lipids.
Anti-microbial peptides produced in response to engagement of various PRRs account for most of the inducible immunity against microbes noted in many invertebrate animals and plants. Although these peptides are probably less central to host immunity in most vertebrates, evidence indicates that they make important contributions to immunity in more highly evolved animals, including mammals. In humans, active anti-microbial peptides, such as the α- and β-defensins, are constitutively or inducibly produced in skin and epithelia of the gastrointestinal and respiratory tracts. These molecules most likely act as natural preservatives of epithelia that are colonized or frequently exposed to microbial flora. Because the acquisition of resistance against these agents by sensitive microbial strains is extremely unusual, anti-microbial peptides are of great interest as templates for the development of new anti-microbial pharmaceuticals.
Influence of Innate Mechanisms on Adaptive Immunity
In addition to functioning as a first line of defense against invading pathogens, a critical feature of the innate immune system in higher animals such as mammals is its effect on activating the adaptive immune system. In fact, it is now clear that in most situations, the adaptive immune system mounts a response to a pathogen only after the pathogen has generated signals via PRRs of the innate immune system. This principle serves as the basis for the adjuvant effect, which is the observation that antibody and T cell responses are efficiently generated against protein antigens only if these are introduced together with a nonspecific activator of the immune system, which is generically known as an adjuvant. Most adjuvants are in fact extracts or products of bacteria, and it is clear that in most or all cases, adjuvant effects result from activation of the innate immune response.
Innate immune responses can prime or potentiate the adaptive immune response in many ways ( Fig. 20.4 ). In the case of T cell responses, one extremely important and well-recognized mechanism involves the upregulation of co-stimulatory molecules. T cells require at least two signals to become activated from a naïve resting state. One signal is provided through the T cell antigen receptor by its binding to a specific peptide ligand presented by a class I or II MHC molecule. The second signal is provided by one of several co-stimulatory ligands that are expressed by specialized antigen-presenting cells such as dendritic cells. The best studied of these are the molecules of the B7 family, B7-1 (CD80) and B7-2 (CD86), which engage the activating receptor CD28 on the surface of the T cell. Expression of B7 family co-stimulatory molecules on the surface of antigen-presenting cells is controlled by the innate immune system, such that these molecules are induced to appear at functional levels only after PRRs, such as members of the TLR family, have been activated by recognition of their cognate PAMPs or DAMPs.
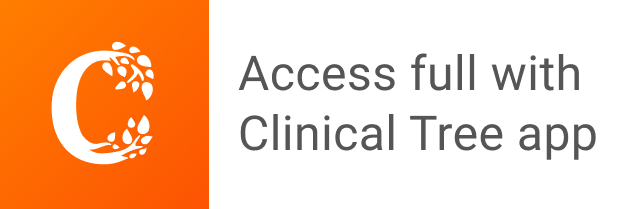