Immunohistochemistry
Immunohistochemistry has an essential role in the evaluation of muscle biopsies and in examining protein localization. The term ‘protein expression’ is often applied to describe immunohistochemical results, but it should be remembered that the technique only reflects localization of a protein, not the related RNA synthesis, and the gene coding for it may not be active at the time the protein is localized. In addition, absence of labelling may sometimes be because the epitope of the antibody is masked and inaccessible. In addition, RNA from a gene may not be translated into protein, but it is the protein that is of pathological significance. Immunohistochemistry is complementary to histology and histochemistry, and the results should not be interpreted in isolation from other morphological studies and the overall clinical picture. Defects in protein localization may identify an abnormality in the gene encoding that protein (a primary defect) or they may be a secondary response to an abnormality in another gene. Immunohistochemical abnormalities related to a primary defect are of particular importance in assessing recessively inherited conditions, where both alleles are mutated. In several dominant conditions, expression from the normal allele may mask any alteration in the mutant allele and labelling of the normal and mutant protein may be indistinguishable; analysis of secondary defects is then particularly important. In some dominant conditions there may be detectable accumulation of protein. Thus, analysis of both primary and secondary abnormalities has an important role in the assessment of biopsies.
With the rapid advances in molecular medicine the number of primary defects that can be identified with immunohistochemistry is growing, and there is a trend towards classifying disorders according to the protein defect (e.g. dystrophinopathy, sarcoglycanopathy, actinopathy, desminopathy, etc.). In this book, however, we have adhered to the classical clinical classification (see Ch. 8 ), as this still forms the basis for clinical diagnosis. This chapter aims to summarize aspects of methodology for immunohistochemistry and also the analysis of proteins relevant to diagnosis, particularly using commercial antibodies that are readily available to everyone (see ). In the last edition of this book we included a table of useful commercial antibodies and their suppliers, but there are now so many sources to choose from that can easily be accessed on the internet we have dispensed with the table. It is important to remember, however, that different companies market the same clone and titres may vary. In the sections on pathological changes we have retained some historical references to reflect the major contribution of so many workers in the field and updated this with more recent studies and reviews.
Many antibodies are now available for studying diseased muscle, and they have widened our understanding of pathological features, but this chapter is not intended as a comprehensive account of the myriad of features that have been identified by the application of antibodies. The objective of this chapter is to give an overview of methods, the use of immunohistochemistry in relation to defective proteins associated with neuromuscular disorders, and to discuss some of the major muscle proteins that are defective in neuromuscular disorders. There is some inevitable overlap with the chapters relating to specific disorders, and there is repetition of some information, but this is included in order to give a more complete overview. This chapter should be read in conjunction with the appropriate disease-related sections.
Methods for Immunohistochemistry
Immunohistochemistry is used to visualize and localize specific protein components of a tissue. The principle of the technique is the specific affinity of an antibody for its antigen. Allied techniques are the labelling of glycoproteins with lectins, the labelling of receptors with a ligand such as a toxin (e.g. the specific affinity of bungarotoxin for acetylcholine receptors at neuromuscular junctions) and labelling of nucleic acids by in situ hybridization. Similar methods of detection and amplification have been developed for all these techniques, but their diagnostic role is currently less than that of immunohistochemistry.
Use of Automated Machines and Immunolabelling Kits
Several companies now market machines for automated imunolabelling of sections, and their use is now widespread. Although these were originally designed for the use of paraffin, fixed sections protocols can be adjusted to work with frozen sections immunolabelled with brightfield markers. Frozen sections are more vulnerable to the procedures of a machine and may lift off or curl, and consistent results may take time to achieve. In addition, the effect of reagents supplied needs to be considered: for example, low concentrations of the detergent triton X-100 are often a component of the kits supplied and, whilst this can reduce background and give excellent results, it must be remembered that it can affect membrane phospholipids. The immunoglobulin class of the antibody used also has to be considered and methods adapted accordingly. Similarly, the dilution of antibodies and the use of commercial detection kits used for labelling both manually and with a machine (e.g. EnVision or Menarini kits) must be empirically determined. Double labelling of myosin isoforms has been successfully achieved on a machine using brightfield markers (see below; ). Multiplex labelling using more than one antibody and different coloured brightfield chromogens or fluorescent labels are being developed for use on automated machines and may soon have a wider use in routine pathological assessment as multiplex labelling using manual methods is informative ( ). It must be remembered, however, that assessment of each antibody separately may also be necessary as any change in colour in the combined signal will be determined by the proportion of each protein detected. Also, the detection levels of fluorochromes can vary in different channels. Double labelling using fluorochromes in more than one channel is a good control for this. We routinely assess collagen VI and perlecan double labelling using both red and green excitation filters for the visualization of both proteins. When performing double labelling, assessment of each protein individually in serial sections or with two different fluorochromes may then be necessary. Each laboratory must determine for themselves the time/cost and efficiency benefits of using a machine and multiplex methods and be aware that technical issues can arise with any method used. The sections below describe manual methods of immunolabelling that we have found satisfactory over the years and factors to consider.
Tissue and Section Preparation for Immunohistochemistry
As for histochemistry, all tissues should be rapidly frozen in isopentane cooled in liquid nitrogen or propane, and immunolabelling performed on cryostat sections, as described in Chapter 1 . If sections are too thick they may ripple and lift off the slides, a problem that can occur with some antibodies but not others. For immunohistochemistry, we find a thickness of 5–7 μm optimal. The epitope for some antibodies is destroyed or masked by fixation, and unfixed frozen sections are then essential. Some antibodies, however, may only give satisfactory results on fixed material, in which case frozen sections can be post-fixed (see below). This is rare for the antibodies currently used for the diagnosis of neuromuscular disorders. If only formalin-fixed, wax-embedded material is available, various antigen retrieval techniques using pre-treatment of sections with enzymes and/or microwaving can be tried. This is useful for archival material but is not the method of choice for routine studies. In our experience, most antibodies for the diagnosis of neuromuscular disorders give good results on unfixed, untreated cryostat sections. Methanol or acetone or the detergent triton, however, is often used.
As described in Chapter 1 , sections collected on Superfrost or Superfrost Plus slides or uncoated coverslips can be stored until required at −20°C, or lower, if wrapped in clingfilm and/or foil. This has the advantage that batches of biopsies can be labelled in parallel, which is time and cost-effective, and each sample then acts as a control for the others, enabling identification of any technical problems. A batch should never consist of samples all with the same provisional diagnosis (e.g. not all potential cases of Duchenne muscular dystrophy: DMD), and a single biopsy should not be labelled in isolation. If results are needed urgently and a batch of cases cannot be assembled, or if additional or repeat studies are needed, a second sample, preferably of normal muscle, should always be included as a control. Sections should be air-dried at room temperature for about 20–30 minutes before use. If only a few sections from a package of stored frozen sections are required, the remaining sections should not be allowed to thaw as condensation may form and freeze when the sections are returned to the freezer, resulting in artefact when the section is subsequently used.
Fixation of Sections
Many antibodies when applied to muscle sections do not require fixation, and this is our method of choice. Acetone or methanol, however, is frequently used for studies of muscle sections, but in our experience this is often unnecessary for the study of human muscle. Formaldehyde or paraformaldehyde, at various concentrations, is also sometimes used. Acetone or methanol, or permeabilization with Triton X-100 (0.05–0.2%) or saponin is often necessary for localization of intercellular antigens in cultured muscle cells and can also be used on sections. A fixative or permeabilization solution may affect the epitope of an antibody and if fixation is required, the most suitable, and its concentration, has to be determined experimentally.
Immunolabelling and Washing
For manual immunolabelling, dry sections are placed in a moist atmosphere to prevent evaporation of the small volumes of reagents, as described in Chapter 1 . Use of a hydrophobic pen to draw round each section is a convenient way to reduce the volume of reagents used and to prevent reagents spreading. Coverslips or slides can be placed in racks and washed collectively in a dish, or they can be rinsed individually. Three rinses of buffer are required, but the timing of each is variable. It can be from 3 to 5 minutes, or in the case of individual coverslips, 10-second dip washes are adequate. The staining tray shown in Figure 1.10 has a magnetic strip which holds the slides in place and is a convenient way to wash a batch of slides by gently expelling buffer from a ‘squeezy’ bottle over the sections for about 30 seconds. Sections must not be allowed to dry out at any stage or false results and artefact will arise. It is also important to ensure that there are no bubbles in the antibody solution when applied, as no labelling will occur under the bubble.
Blocking Agents
Non-specific background, which reduces the signal-to-noise ratio, may be a problem. Additives to the buffer, such as bovine serum albumin (BSA; 2%) or detergents (0.2% Triton X), or normal serum from the same species as the secondary antibody, can be used to reduce non-specific binding of secondary antibodies but, as with fixatives, they may affect the epitope of the antibody. Some tissue components may contain endogenous enzymes, such as peroxidase or alkaline phosphatase. For example, macrophages contain endogenous peroxidase and blocking agents are needed if cluster of differentiation (CD) markers are localized with peroxidase. Endogenous peroxidase can be blocked with hydrogen peroxide (1%) and endogenous alkaline phosphatase can be blocked with levamisole (1 mM), although the EnVision kit contains a peroxidase blocker. It can sometimes be useful to observe endogenous peroxidase activity and to know macrophages are present without having to use specific cell markers (see Fig. 6.8 ). Endogenous biotin can be blocked by applying unconjugated avidin followed by biotin, available as a commercial kit. We find that there is rarely an advantage in using blocking agents for the study of human muscle; good washing and optimal dilution of the antibodies are more critical factors. Phosphate-buffered saline (0.1 M or 0.2 M) at pH7.2–7.4 is usually used for washing, and commercially available tablets are now a convenient way to make this up.
Primary Antibodies
A wide variety of primary antibodies to muscle proteins are now commercially available, in particular those that detect primary defects in recessive muscular dystrophies. Several companies sell relevant primary antibodies, and most companies now have a website. There are also various directories and hybridoma banks (see Appendix 2 ). Several companies market the same antibody clone, so purchasing an antibody from a different company does not necessarily mean it is a different antibody. Attention should be paid to the characterization of a commercial antibody as this is not always complete on data sheets, and also to the recommended storage of an antibody. If freezing is recommended, an antibody should be divided into aliquots of convenient volume to avoid repeated cycles of freeze–thawing. Company websites (e.g. Abcam) offer useful advice on storage and the length of time that an antibody can be used, which is increasingly important for laboratory accreditation. When possible, characterization of an antibody should be checked in the original publication describing the use of the antibody, or the information obtained from the company. Dilution of a primary antibody should always be assessed by titration in each laboratory, and is dependent on such factors as supplier, poly- or monoclonal antibody, secondary antibody, time and temperature of incubation, and the detection system used. As for washing, phosphate-buffered saline at pH7.2–7.4 is used for diluting antibodies. Incubation times can vary from 30 to 60 minutes to overnight at 4°C. The latter sometimes reduces background and lower dilutions of antibody may be possible. Both monoclonal and polyclonal antibodies are available. The former, released from hybridoma cells, recognize a single epitope and are highly specific. Mouse monoclonal antibodies have been used for many years but rat and rabbit monoclonal antibodies are also now available. Polyclonal antibodies are usually purified from serum following injection of the antigen into an animal species (e.g. rabbit, goat or sheep) and recognize several epitopes on the antigen. A control not using the primary antibody is particularly important when using polyclonal antibodies (see below). Double labelling using primary antibodies raised in different species is relatively straightforward, but if the primary antibodies are both monoclonals from the same species kits are available (e.g. Zenon immunolabelling kits). For double/multiplex labelling it is often possible to use a cocktail of antibodies applied at the same time, provided they do not interfere with each other, and appropriate secondary antibodies then applied for each. If multiple antibodies are applied sequentially, the saturation of the first primary must be considered and any possible binding of the unsaturated first primary antibody with subsequent secondary antibodies used.
Detection Systems
It is common to use an indirect detection method to visualize the primary antibody, rather than directly labelling each primary antibody with a marker, and several commercial kits are now available. Indirect labelling gives more flexibility as it involves applying a secondary antibody against the appropriate immunoglobulin of the species in which the primary antibody was raised, and the same one can then be used to visualize several different primary antibodies. Most antibodies are immunoglobulin G (IgG) immunoglobulins but some are IgM. It is essential to use a secondary antibody to the appropriate immunoglobulin or to use one that recognizes all classes. Secondary antibodies to different IgG classes can be useful for multiplex labelling ( ). The secondary antibody may be directly conjugated to biotin, an enzyme or a fluorochrome. Biotin conjugates are then followed by streptavidin labelled with a marker of choice. This amplification technique has the advantage of enhancing the signal because of the total number of bound avidin molecules. Streptavidin directly labelled with a fluorochrome or a preformed avidin–biotin complex (ABC) may be used. Good enhancement is also achieved with commercial kits (e.g. EnVision or Menarini) and the tryamide system (Zenon immunolabelling technology) in which peroxidase is used to catalyze the deposition of biotin or fluorochrome-labelled tryamide close to the antigen–antibody binding site. The deposited biotin is then visualized with streptavidin conjugated to peroxidase or a fluorochrome.
The choice of label to visualize the antibody is often a matter of personal preference but it is also governed by the type of microscope available, the need for a permanent preparation, the amount and localization of the antigen and the affinity of the antibody used. Enzyme labels, such as peroxidase or alkaline phosphatase, provide permanent results and it is easier to see the overall structure of the tissue, particularly if sections are counterstained after immunolabelling. With fluorescent labels, however, it may be easier to distinguish small areas of localized antibody which appear bright against a dark background, and specificity can easily be checked by changing the excitation filter. Methods for quantifying fluorescent signals are also being developed (see below). Aqueous mountants have now improved which reduce fading, and fluorescence can be retained for several weeks, months or sometimes even years. Good fluorescent nuclear counterstains are also now available (see below). Fluorescent methods are usually quicker to apply and avoid the use of hazardous substances such as diaminobenzidine (DAB). Double and multiplex labelling is easier using fluorochromes and the relationship of different antigens closely localized at the same site is more precisely determined; however, brightfield double labelling is also being used ( ). Illustrative examples of the use of both peroxidase and fluorochromes are given in this book.
Fluorescent Labels
Several fluorochromes are now available, such as fluorescein, rhodamine, Texas Red, AMCA, Cy3 and Cy5 and Alexa probes. The choice is often one of personal preference, but factors such as cost, intensity, filters fitted to the microscope and fading also need to be considered. Fluorescein isothiocyanate (FITC) fades rapidly on excitation and FITC–Ig conjugates bind non-specifically to muscle components. Its use is diminishing in favour of Cy and Alexa probes (marketed by Molecular Probes) which give a particularly high signal-to-background ratio and are resistant to fading on storage and with excitation. Bleaching of sections can occur when high-power objectives are used. Storage of the labelled sections in the dark and at 4°C helps to reduce fading. Autofluoresence in muscle, such as that associated with blood vessels and lipofuscin, should be identified by exchanging excitation filters. The development of new labels encourages users to change their methods. While this has obvious advantages, pathological comparisons of intensity are important and new baselines may have to be established if changes are made. Our method of choice was previously a biotin–streptavidin–Texas Red system but we now routinely use streptavidin–Alexa 594 or 488 when labelling by hand with fluorescent markers, particularly for localizing small areas or when double labelling is required. For the majority of antibodies we now routinely use a peroxidase-based method on a Ventana (Roche) Discovery Ultra or a Dako Autostainer machine. Methods have had to be adapted and reagents varied in order to obtain good reproducible results with some antibodies.
Enzyme Labels
Secondary antibodies can be conjugated to an enzyme that can then be histochemically detected at the site of the antigen by deposition of a coloured, insoluble reaction product. Peroxidase detected with DAB has traditionally been the most used enzyme label. It produces a brown end product which is stable for long periods at room temperature and can be enhanced with silver or nickel to produce a black end product. Other substrates that can be used include aminoethyl carbazole (red end product) or 4-chloro-1-naphthol (blue end product). A common alternative to peroxidase is alkaline phosphatase, which, with the appropriate substrate, can be visualized as a red, blue or black end product. Glucose oxidase and β-galactosidase are also useful, particularly for double labelling of two different antibodies. Amplification, as mentioned previously, can be obtained with a biotin–avidin system, either with a preformed complex, directly conjugated to streptavidin alone, or various commercial kits. Peroxidase antiperoxidase also provides enhancement but is now less commonly used since the wider availability of biotinylated systems. As with fluorochromes it is necessary to establish baselines and become accustomed to levels of intensity of peroxidase labelling with each antibody used. It is important to know how a particular antibody works in your laboratory with your method.
Counterstaining and Mounting
Counterstains provide an overall impression of the morphology of the tissue and can be nuclear or cytoplasmic—more commonly the former. They are not essential, and their use is one of individual choice. In sections where labelling is expected to be absent, such as with dystrophin in DMD or mature muscle with no immature myosin isoforms, a nuclear counterstain can be helpful. In Emery–Dreifuss muscular dystrophy the absence of emerin from the nuclei using a peroxidase detection system is easier to assess without a counterstain. With fluorescence, however, a nuclear counterstain at a different wavelength to the one used to detect emerin can be helpful to show where the nuclei are. The choice of counterstain is dependent on the colour of the end product or fluorochrome. Haematoxylin and methyl green are common nuclear counterstains used after peroxidase. If too much haematoxylin is present, it may give a pale delineation of the sarcolemma and care is then needed in assessing any very low levels of sarcolemmal proteins. For example, when assessing dystrophin in peroxidase-labelled sections from cases of DMD the low levels of minor transcripts may be obscured or low levels of sarcolemmal major histocompatibility complex (MHC) class I may be difficult to identify if the haematoxylin counterstain is too strong. Fluorescence is then an advantage, as the low levels are visible against the dark background. For fluorescence, the nuclear counterstains most commonly used are 4′6-diamidino-2-phenylindole.2HCl (DAPI) (1 μg/mL), Hoechst dye (10 μg/mL), which are both blue under ultraviolet light, or ethidium bromide (1 μg/mL) or TOTO, which are red (all available from Molecular Probes). Mountants containing a counterstain are also commercially available (e.g. with DAPI from Vector Laboratories).
The choice of mountant is also determined by the visualization marker. Sections labelled with enzymes such as peroxidase can be dehydrated in graded alcohols, cleared and mounted in synthetic resins. Fluorescent markers require aqueous mountants and several commercial ones contain antifading agents. We have found Hydromount (National Diagnostics) to be useful as this dries to hold the coverslip in place and fluorescence lasts for several weeks or months. Mountants from Vector Labs are also useful. If a glycerol-based mountant is used, which does not dry, the coverslip should be sealed and held in place by nail varnish.
Baselines for Interpretation
Interpretation of results is dependent on several aspects, including the visualization technique used, appropriate controls and maturity of the fibres. Results vary between different laboratories, even when using the same antibodies with the same detection conditions, and it is important that each laboratory establishes optimal conditions and baselines for each antibody and knows how a particular antibody works in their hands. Controls are essential to check for non-specific background and to check for preservation of the tissue. An important control to check for non-specific labelling is omission of the primary antibody so that sections are only labelled with the secondary antibody and the detection reagents. Necrotic fibres often contain IgG, which is detected by an anti-IgG secondary antibody and must not be confused with specific labelling.
Immunohistochemical assessment of the sarcolemma is a fundamental aspect of diagnostic muscle pathology, and it is essential to know if the membrane is well preserved. Both the plasmalemma and basal lamina may be damaged or lost if the tissue is affected by freezing artefact or in pathologically damaged fibres. Sarcolemmal proteins may also be lost or reduced in postmortem material, depending on the time from death to freezing the sample. The basal lamina is more resilient than the plasma membrane and may be retained on some necrotic fibres and fibres in postmortem material, even though the plasma membrane is lost ( Fig. 6.1a, b ). Plasma membrane proteins are sometimes less apparent on regenerating fibres ( Fig. 6.1c, d ), and sometimes labelling within a regenerating fibre is seen with some antibodies. Studies of dystrophin and all plasma membrane proteins should always be accompanied by parallel studies of β-spectrin, as these give a good indication of the overall preservation of the sample. Antibodies to caveolin-3 can also give a good indication of membrane integrity, and labelling is only pathologically affected in a few situations (see below). Similarly, any studies of basal lamina proteins should be controlled with studies of a laminin chain or a basement membrane collagen, depending on the likely nature of the disorder in question. We routinely use an antibody to laminin γ1 to assess preservation of the basal lamina as, to date, no pathological immunohistohistochemical abnormalities in its localization have been observed in skeletal muscle. For assessing components of the basement membrane, such as collagen VI, we use perlecan or collagen V.

Variation in fibre size is often more apparent in sections immunolabelled with antibodies to sarcolemmal proteins. We therefore routinely study the sarcolemma to assess this and tissue preservation, even if a defect is not suspected.
Developmental Regulation of Proteins
It is important to know the localization patterns of an antibody in mature, neonatal and fetal muscle, as many proteins are developmentally regulated and expression alters with age. When studying an antibody to a protein that has not previously been studied in your patient cohort, it is often useful to study its localization in fetal, neonatal, dystrophic (e.g. DMD) and normal muscle and a range of neuromuscular disorders to get an indication of specificity and how expression varies during development. In addition, a different isoform of a protein may be present at different stages of development. This is particularly important when assessing samples that may contain regenerating fibres, or samples from neonates. We summarize here our own experience with the panel of antibodies we most often use for diagnosis. This is not intended as a comprehensive account of the developmental regulation of muscle proteins but reflects developmental aspects with the antibodies used for diagnostic assessment.
Some proteins change their isoform during maturity ( Table 6.1 ). Studies of developmental isoforms of myosin are useful for assessing immaturity and to help distinguish an atrophic fibre from one that is small because it is regenerating. Our studies of regenerating canine muscle showed that an immature isoform of myosin is detectable for a longer length of time than other developmentally regulated proteins such as utrophin, desmin and neural cell adhesion molecule (NCAM) ( ; unpublished observations). For studies of human muscle we routinely use antibodies to developmental (Leica/Novocastra MHCd) and fetal (Leica/Novocastra MHCn) myosin heavy chain to assess maturation (see Fig. 6.1 ) as we have found these useful for assessing immature and regenerating fibres in dystrophic muscle. MHCd probably detects the embryonic myosin isoform and highlights the small basophilic regenerating fibres ( ) (see also Table 6.4 ). It is present in myotubes at the early stages of regeneration round necrotic fibres ( Fig. 6.2a, b ) but usually only a few larger fibres in dystrophic muscle. It is not clear if MHCd recognizes the embryonic isoform encoded by the MYH3 gene, but it is assumed to, and the terms ‘embryonic’ and ‘developmental’ are often used synonymously. The antibody marketed by Leica (MHCd) was raised against rat neonatal muscle, but F1.652 to embryonic myosin from the Developmental Studies Hybridoma Bank (DSHB) gives very similar results (see Fig. 6.24 ) The MHCn antibody detects an isoform that is present in human fetuses from at least 7 weeks’ gestation, and in addition to regenerating fibres of variable size it labels several other fibres in dystrophic muscle and round necrotic fibres (see Figs. 6.2 and 6.24 ). This is probably the isoform encoded by the MYH8 gene. As this isoform is abundant in human fetal muscle, we refer to this as ‘fetal myosin’, but the maturation of muscle in other species, such as mice and rats, is later and at birth immature myosins are abundant and often referred to as ‘neonatal myosin’. The MHCn antibody, however, does not recognize fetal myosin in all species (e.g. not in dog or mouse). MHCn labels a population of fibres in human neonatal muscle, but this number declines with age. A large number of positive fibres are seen at birth and relatively few after 4–6 months of age, but the normal spectrum of expression is not fully established as all samples studied have been taken for a clinical reason and normality cannot be established beyond doubt. A few positive fibres may be seen up to 1–2 years of age, but it is not yet clear whether this is within the normal spectrum of expression or if it is pathological. Our assessment of biopsies with no, or minimal, pathological features, however, indicate that developmental myosin is down-regulated by birth and fetal myosin by 4–6 months (Sewry, Feng unpublished results); thus, fibres with fetal myosin in patients older than 1 year of age probably reflect an abnormality. Patients with developmental delay, however, may show several fibres with both these isoforms. The presence of fetal myosin is often interpreted to reflect immaturity, but in some situations the presence of immature myosin isoforms is confusing, as there is evidence from studies on animals that they can be re-expressed in denervated muscle and during ageing ( ). In human muscle, some small fibres in motor neurone disease and the groups of small fibres in spinal muscular atrophy, both of which are thought to be non-innervated, express fetal myosin and sometimes additional developmentally regulated proteins ( ; see also Ch. 9 ). It is not known if this could reflect regeneration induced by denervation, and/or arrest in maturation and/or the re-expression of developmental and fetal myosin induced by the pathological state. Nuclear clumps which are chronically atrophic fibres also express fetal myosin but not developmental myosin ( Fig. 6.2c ; ). Thus, labelling with MHCn is not only a feature of immaturity but can also be a consequence of abnormality, which cannot always be defined. Nevertheless, studies of fetal myosin are important for the assessment of other developmentally regulated proteins and for identifying different patterns of expression that are associated with different disorders (see below). The antibodies to developmental/embryonic myosin (MHCd and F1.652) are useful for recognizing the early stages of regeneration but, like fetal myosin, this isoform can also be induced by events such as denervation.
Change of Isoform |
Actin cardiac → skeletal Myosin embryonic → fetal → fast or slow |
Low Expression on Immature and Regenerating Fibres |
β-Spectrin round some small regenerating fibres C-terminal dystrophin (sometimes) Some dystrophin-associated proteins Neuronal nitric oxide synthase (nNOS) Laminin β2 extrajunctional labelling weaker in neonates Integrin α7 extrajunctional labelling weaker in neonates |
High Expression on/in Regenerating Fibres ∗ |
Embryonic (developmental) and fetal myosin heavy chain Utrophin Laminin α5 Neural cell adhesion molecule (NCAM) Vimentin Desmin Major histocompatibility complex (MHC) class I |























Satellite cells that give rise to regenerating fibres can be identified with antibodies to various proteins that include Pax7, CD34, caveolin-1, calcitonin, M-cadherin, nestin and NCAM and their position under the basal lamina visualized with an antibody to a laminin chain or collagen IV. The availability of commercial antibodies to these proteins and their use in different species vary. Satellite cells in human muscle have different properties than those in animals such as mice, and their number is influenced by age, sex, exercise, fibre type and the muscle studied as well as the pathological state and whether they are activated ( ). NCAM, detected by the Leu-19 antibody, detects activated satellite cells and these can be seen in varying numbers in different neuromuscular disorders (see Fig. 6.15d ; ). As many biopsies from young children with no or minimal pathology do not show satellite cell-like areas positive for NCAM, we conclude that NCAM in human muscle recognizes only activated satellite cells, in addition to regenerating fibres and denervated fibres. Several cell types in addition to satellite are thought to have a role in regeneration but they have not been extensively studied in human pathological biopsies ( ).
Muscle differentiation, development, growth and regeneration, including satellite cell function, are dependent on a family of basic-helix loop-helix myogenic regulator factors which includes MyoD, Myf5, myogenin and MRF4 ( ). Recently mutations in some of the genes that encode for these factors such as Myf5 and also PAX7 have been associated with a myopathy (D ; ). Patients homozygous for Myf5 mutations have a disorder characterised by ophthalmoplegia, scoliosis and rib abnormalities ( ). Myf5 was reduced in muscle from patients with variants in PAX7 and biopsies showed small fibres and excess fibro-adipose tissue. The clinical phenotype in these patients included ptosis, hypotonia scoliosis and facial dysmorphism ( ).
Another important muscle protein that changes isoform during development is actin . Two isoforms have been identified that differ by only four amino acids at the N-terminal region ( ). High salt is required in the buffer to specifically demonstrate cardiac actin with the commercial antibody that we have used. In fetal skeletal muscle the form found in adult cardiac muscle is predominant, and this is then replaced by the isoform of skeletal muscle. The cardiac isoform is present in the small, basophilic regenerating fibres in dystrophic muscle (and sometimes some larger fibres), and only a few positive fibres are detected at birth, with most being lost by the late stages of gestation ( ). All intrafusal fibres of spindles show cardiac actin but variable fetal, fast and slow myosin (see Fig. 6.31 ).
It is important to assess the maturity/immaturity of muscle fibres in neonatal muscle and when regenerating fibres may be abundant. Some proteins are more highly expressed in immature muscle; others are lower (see Table 6.1 ), as judged by antibody immunolabelling. Expression studies at the RNA level of many of these proteins are limited. Utrophin , the autosomal paralogue of dystrophin, is high on the sarcolemma of fetal muscle fibres and on regenerating fibres ( Fig. 6.3a, c ). High internal labelling of regenerating fibres may also be seen. Utrophin and dystrophin are both expressed at the sarcolemma from at least 9 weeks’ gestation, and sarcolemmal labelling of utrophin peaks at about 20 weeks’ gestation ( ). After about 26 weeks’ gestation and in mature muscle utrophin is no longer seen on the sarcolemma, and it is confined to blood vessels, nerves and neuromuscular junctions. Utrophin has also been reported at myotendinous junctions, but it is not abundant at this site. In the absence of dystrophin, or reduced dystrophin, as in Duchenne and Becker muscular dystrophies, and in inflammatory myopathies, sarcolemmal utrophin is prominent ( Fig. 6.3b ; ). Some sarcolemmal utrophin may also sometimes be seen in other disorders ( ). The assessment of utrophin must take account of immaturity of the fibres by reference to the presence of developmental and fetal myosin so that only the abnormal overexpression in mature fibres is considered. Low levels of sarcolemmal utrophin may occur in neonatal muscle that is not dystrophic and is not related to the presence of fetal myosin ( ). The reason for this is unknown. It can also be seen on fibres adjacent to some tumours, which raised the possibility that factors such as cytokines from the tumour or inflammatory cells might up-regulate utrophin ( ).
Laminin α 5 and MHC class I (MHC-I) are also proteins that are high on regenerating fibres, and assessment of their pathological presence again must be related to fetal myosin ( Fig. 6.3b, d ). Laminin α5 is present on fetal fibres and its presence declines during fetal development ( ; see Fig. 6.9 ), but moderate sarcolemmal labelling may be present in neonatal muscle. MHC-I, however, is not present on the sarcolemma of fetal muscle fibres, but low levels can be a non-specific finding in neonatal muscle and a variety of disorders (see below). All basal lamina proteins may appear to be high on regenerating fibres because of duplication of the basal lamina.
Vimentin and desmin are also high in fetal and regenerating fibres. Both are down-regulated during development; vimentin is only detected on vascular tissue in mature muscle, and desmin is seen at the sarcolemma and internally in the fibres at the level of the Z line but accumulates in some pathological situations (see Chapter 15, Chapter 16 ).
The neural cell adhesion molecule (NCAM; CD56) is present on the sarcolemma of regenerating, non-innervated fetal fibres, activated satellite cells (see earlier), nerves and at neuromuscular and myotendinous junctions. Both sarcolemmal and internal labelling of fibres is seen (see Fig. 6.15c ). Once a fibre becomes innervated, NCAM becomes localized to the neuromuscular junction. Non-innervated regenerating fibres with extrajunctional NCAM also express fetal myosin but, as pointed out previously, some small fibres in neurogenic disorders express fetal myosin. NCAM becomes extrajunctional following denervation ( ), so, with the presence of both these proteins, and others associated with immaturity, it can be difficult to distinguish a denervated fibre from a regenerating fibre. Extrajunctional NCAM is a common feature in DMD, but the origin of this in relation to regeneration and innervation is not clear. Similarly, in patients with mutations in the ECEL1 gene, several fibres with NCAM but without fetal myosin may be present which may relate to a neural problem ( ). The association of NCAM with innervation is the traditional understanding of its role, but NCAM is also thought to be a sensitive marker for cellular sialic acid levels and its role in sialylation has been studied in GNE myopathy but not a wide variety of disorders ( ). Neuronal nitric oxide synthase (nNOS) is absent from the sarcolemma of denervated fibres ( ), and the combination of comparisons of fetal myosin, nNOS, NCAM and laminin α5 (which is not usually high on denervated fibres) can be informative in distinguishing immature fibres from denervated fibres. Fibres without both fetal myosin and nNOS are likely to be denervated (see Ch. 9 ).
Other proteins are only weakly labelled in immature muscle. As accompanying RNA studies have not been done, the possibility that any apparent weak or reduced immunohistochemical labelling relates to masking of an epitope of a particular antibody rather than to low expression cannot be excluded. Low immunolabelling may also relate to the affinity of a particular antibody. Several sarcolemmal proteins, such as β-spectrin, sarcoglycans, C-terminal dystrophin, integrin α7 and nNOS, may appear weak on some small regenerating fibres that have fetal myosin, possibly because of immaturity and a plasma membrane that is not fully developed (See Figs 6.4 and 6.8 ). Internal labelling of small, basophilic fibres may be seen with some antibodies (e.g. caveolin-3;).
Labelling of neonatal muscle with some antibodies is also weak and may make pathological assessment difficult at this stage. For example, sarcolemmal labelling of integrin α7 is low in immature muscle, and nNOS with the antibody from Santa Cruz that we have used (which is no longer available) is often very weak or absent in patients under 1 year of age ( ). Labelling of nNOS at the neuromuscular junction in immature muscle, however, is prominent. We have also noticed this weak sarcolemmal labelling in neonatal muscle with antibodies to laminin β2, which, in addition to the high expression at the neuromuscular junction ( ), is also very prominent extrajunctionally and increases in intensity with age ( ; Sewry unpublished observations). At birth, blood vessels are more intensely labelled with laminin β2 than the sarcolemma, but by about 3 years of age the intensities are usually comparable (see Fig. 6.9 ).
Use of Tissues Other Than Muscle
Although muscle is the tissue of choice, useful diagnostic information on some proteins can be obtained from other tissues. For example, skin expresses many of the extracellular matrix proteins found in muscle, and skin biopsies are a useful alternative to muscle for studying laminin α2 and collagen VI, particularly when muscle wasting is extensive or muscle is unavailable ( ; see Ch. 12 ). Changes in the localization of the α5 and α6 chains of collagen VI have also been shown in skin in cases of Ullrich congenital muscular dystrophy ( ). Skin biopsies are also useful for studying the expression of plectin as the basal keratinocytes at the epidermal–dermal junction show a reduction or absence in epidermolysis bullosa with muscular dystrophy ( ). Similarly, emerin, which is expressed in the nuclei of many tissues, can easily be assessed in skin and oral foliate cells ( ; see Ch. 13 ). Caution is needed using the latter, however, to ensure that the sample does not contain dead buccal cells in which the nucleus does not label.
Prenatal diagnosis can be aided by studies of the immunolabelling of proteins in chorionic villi: for example, in congenital muscular dystrophy caused by a primary deficiency of the laminin α2 chain—‘merosin’-deficient congenital muscular dystrophy (congenital muscular dystrophy type 1A or MDC1A) ( ; see Ch. 12 ) or the Ullrich form of congenital muscular dystrophy caused by mutations in collagen VI ( ).
Cultured fibroblasts from skin biopsies are of use for some immunohistochemical studies and can be useful for mitochondrial and metabolic studies. Research suggests that fibroblasts are useful for studying collagen synthesis and glycosylation, including using flow cytometry ( ). Fibroblasts can also be transfected with MyoD to convert them to muscle cells for the analysis of muscle specific proteins but this is not widely used for diagnosis. It is likely that the use of tissues other than muscle will increase in the future as more biomarkers are identified. Blood monocytes may also be of use if they express a particular protein. Immunoblotting of dysferlin from monocytes suggests this approach is also useful ( ). Recently an immunoassay for dysferlin in neutrophils has also been developed (Cox et al 2019).
Pathological Features of Diseased Muscle
In this section we give an overview of the immunohistochemical patterns in diseased muscle in relation to primary and secondary defects. This section should be read in conjunction with the appropriate chapters on individual disorders (cross-referenced). Many of the primary defects relate to recessively inherited conditions. Many new gene defects have been identified and the localization of the affected protein studied. We discuss in this section defects in the more commonly affected proteins which generally only show an abnormality when the mutations are recessively inherited. In dominant conditions immunohistochemical abnormalities of a protein are more difficult to detect, as the normal allele produces a normal protein product whose localization is often normal. Secondary abnormalities then have an important role. Protein accumulation, however, can occur in some dominant conditions, both as a primary and secondary effect. Secondary changes are also important in recessive conditions when studies of the primary protein may show equivocal results or when suitable antibodies to the gene product are not available.
Primary Defects
The number of abnormal primary protein defects and available antibodies that can be detected in neuromuscular disorders by immunohistochemistry is rapidly growing. The proteins listed in Table 6.2 are those that a pathologist needs to consider and to correlate with the clinical phenotype, but the list is not exclusive. Although several new genes responsible for a neuromuscular disorder have been identified, not all have been studied by immunohistochemistry, either because antibodies are unavailable or because they are unsuitable for studies of sections. Immunoblotting (see below), however, can sometimes highlight a defect.
Absence of a Protein | |
Dystrophin | Xp21 muscular dystrophies |
Sarcoglycans | Limb-girdle muscular dystrophies 2C–F |
Dysferlin | Limb-girdle muscular dystrophy 2B |
Caveolin-3 | Limb-girdle muscular dystrophy 1A, rippling muscle disease, hyperCKaemia |
Telethonin | Limb-girdle muscular dystrophy 2G |
Laminin α2 | MDC1A (‘merosin’-deficient congenital muscular dystrophy) |
Collagen VI | Ullrich congenital muscular dystrophy (usually no detectable change seen in Bethlem myopathy) |
Integrin α7 | Mild congenital dystrophy/myopathy |
Emerin | X-linked Emery–Dreifuss muscular dystrophy |
SERCA 1 | Brody disease |
Plectin | Epidermolysis bullosa with muscular dystrophy; limb-girdle dystrophy 2Q |
LAMP-2 | Danon disease |
Mitochondrial complex I | |
(Calpain-3 can be detected on sections and absence seen in limb-girdle dystrophy 2A but it is better assessed on immunoblots) | |
Accumulation of a Protein | |
Actin | Congenital actin myopathy/nemaline myopathy |
Myosin | Myosin storage myopathy |
Myotilin | Myotilin-related myofibrillar myopathy |
Desmin | Desmin myopathy |
VCP | Inclusion body myopathy with Paget disease and frontal dementia |
Membrane-Associated Proteins
Several defective proteins associated with the sarcolemma are responsible for various forms of muscular dystrophy. The gene encoding dystrophin was cloned in 1987 and was the first gene defect to be identified in a neuromuscular disorder (see Ch. 10 ; ). Mutations in the dystrophin gene on chromosome Xp21 cause Duchenne or Becker muscular dystrophy (see ). Dystrophin is a cytoskeletal protein, and immunolabelling of normal muscle shows it is localized to the sarcolemma of every fibre ( Fig. 6.5a ). Abnormal labelling is seen in Xp21 conditions. A secondary reduction, however, can occur in some limb-girdle dystrophies and congenital muscular dystrophies (see below). The majority of cases of DMD show an absence of dystrophin from most fibres or a very marked reduction ( Fig. 6.5b ; see Ch. 10 ). This is thought to be due to disruption of the reading frame by the mutation. Isolated fibres, or small clusters, may show a normal intensity and are known as revertant fibres ( Fig. 6.5c ). These are fibres in which the reading frame has been maintained by skipping the mutation. In Becker muscular dystrophy the reading frame is also maintained and labelling is usually of reduced intensity on all fibres, or uneven on many fibres ( Fig. 6.5d ; see Ch. 10 ). In some Becker muscular dystrophy cases, however, dystrophin immunolabelling may be indistinguishable from normal and, then, assessment of secondary changes is useful. Abnormalities related to a defect in the dystrophin gene can also be seen in manifesting female carriers of DMD ( Fig. 6.6 ) and occasionally in Becker muscular dystrophy carriers ( ), because of random X-inactivation patterns (see Ch. 10 ).
Dystrophin is a large protein, and it is important to use more than one antibody for assessment to avoid false results. If the epitope of an antibody lies within a region of the protein encoded by a deleted region of the gene, dystrophin will not be detected, giving the impression of DMD. If the reading frame is maintained, however, other domains including the C-terminal region will be present, consistent with Becker muscular dystrophy. It is therefore important when assessing a case of muscular dystrophy to use antibodies that recognize N- and C-terminal regions of dystrophin, and it is usual to also include one to the rod domain of the protein. Although the majority of cases of Duchenne and Becker dystrophy conform to the frame shift hypothesis, there are exceptions, and clinical severity should not be judged from dystrophin immunolabelling (see Ch. 10 ; ). Several commercial monoclonal and polyclonal antibodies are available, but their affinity is variable, and caution in comparing results is then needed.
Dystrophin is associated with complexes of proteins that collectively have been called the dystrophin-associated proteins (DAPs) ( ). All of these localize to the sarcolemma in immunohistochemical analysis. Some of these proteins are glycosylated, some are transmembrane or extracellular; others are intracellular. The DAPs are thought to provide a structural link between the subsarcolemmal actin cytoskeleton and the extracellular matrix, stabilizing the membrane during repeated contraction and relaxation. The complex may also stabilize various receptors and ion channels. The DAPs are grouped into subcomplexes ( Fig. 6.7 ): α- and β-dystroglycan, which are post-translational products of the same gene, the sarcoglycans (α, β, γ, δ) and sarcospan, the syntrophins, dystrobrevin and nNOS ( ). Two additional genes with homology to sarcoglycans have also been identified (ε and ζ), and their proteins also localize to the sarcolemma ( ), but they are part of different complexes with roles in other locations, including the neuromuscular junctions, retina and brain ( ). ε-Sarcoglycan is homologous to α-sarcoglycan and is thought to replace it in smooth muscle, where it is closely associated with β- and δ-sarcoglycan ( ). Mutations in ε-sarcoglycan cause myoclonus-dystonia syndrome ( ), but no pathogenic mutations in ζ-sarcoglycan in humans have been found. Defects in the genes for α-, β-, γ- and δ-sarcoglycan have been identified in forms of limb-girdle muscular dystrophies (see Ch. 11 ). Rare mutations in the gene encoding dystroglycan ( DAG1 ) have been identified and are responsible for a form of limb-girdle muscular dystrophy ( ), and rare cases with a congenital muscular dystrophy phenotype have been reported (see Chapter 11, Chapter 12 ; ). The major defect associated with dystroglycan is the secondary post-translational modification of α-dystroglycan by glycosylation, and it is recognized as an important pathogenic mechanism in several forms of congenital muscular dystrophy (see below and Ch. 12 ). The syntrophins are believed to be important in anchoring ion channels ( ), but no mutations in the genes encoding syntrophins, dystrobrevin or nNOS have yet been found, only secondary alterations in their expression (see below).
The sarcoglycans (α, β, γ, δ) function as a group, so that a defect in one results in reduced expression of all four. Complete absence of one sarcoglycan, or the most severe reduction, is usually an indicator of the defective gene and helps to direct molecular analysis ( Fig. 6.8 ). The other three sarcoglycans then show variable degrees of abnormal immunolabelling, ranging from pronounced to minimal. An absence of the whole sarcoglycan complex may occur and is usually associated with a defect in the β-sarcoglycan gene ( ). Immunoanalysis, however, does not always predict the genotype ( ). A secondary reduction of sarcoglycans occurs in DMD (see below).
Other forms of limb-girdle muscular dystrophy are caused by mutations in other genes coding for proteins localized to the sarcolemma, and a reduction can be detected with immunohistochemistry. An absence or marked reduction of the plasma membrane protein dysferlin can be seen in some cases of limb-girdle 2B dystrophy and Miyoshi myopathy. Dysferlin is believed to be involved in membrane fusion and repair ( ). Currently available commercial antibodies do not always give a clear result with immunohistochemistry, and secondary changes in dysferlin expression can also occur in other muscular dystrophies (see below). Abnormalities in dysferlin expression are often clearer on immunoblots, and several proteins should be examined simultaneously to ensure the reduction is not secondary to a primary defect in another protein such as calpain-3 or caveolin-3 ( ).
Caveolin-3 is the muscle-specific form of caveolin, and defects in its gene occur in a dominant form of limb-girdle dystrophy (LGMD1C), in rippling muscle disease, in association with exercise intolerance and rhabdomyolysis and some rare cases with only elevated serum creatine kinase (CK) (see Ch.11 ; ). Caveolin-3 is a plasma membrane protein, and in normal muscle it is seen localized to the sarcolemma. An absence or reduction of the protein can be detected by immunohistochemistry and is a rare example of a detectable reduction of protein resulting from a dominant mutation. Patients with an autoimmune, non-genetic form, of rippling muscle disease have also been identified and muscle biopsies show a mosaic pattern of caveolin expression on muscle fibres ( ).
Extracellular matrix proteins have been shown to have a major role in neuromuscular disorders. Defects in the LAMA2 gene encoding the laminin α 2 chain of merosin (laminin 211) occur in the severe MDC1A form of congenital muscular dystrophy (‘merosin-deficient’ congenital muscular dystrophy; ; see Ch. 12 ). In normal muscle the laminin α2 chain is localized to the basal lamina of every muscle fibre. It is not present in the basal lamina round blood vessels of skeletal muscle, nor of smooth muscle, although a thin layer of labelling of the inner layer adjacent to the lumen of large vessels may be seen and may relate to the tunica intima or inner endothelial layer. This is in contrast to the laminin α5, β1, β2 and γ1 chains ( Fig. 6.9a–e ; and see Fig. 6.32 ) and to blood vessels of the brain ( ). Laminins are heterotrimers, the three chains of which are arranged in a cruciform shape and encoded by separate genes. To date, five α, four β and three γ chains have been identified, which are assembled into at least 15 different forms with a variable tissue distribution ( ). Early nomenclature referred to the chains as A, B1, B2 but this was revised to accommodate the growing number of different chains, and a further revision is now often used ( ). The main variants localized to the sarcolemma are laminin-2 (often referred to as merosin; α2-β1-γ1 chains, also referred to as 211) and laminin-4 (S-merosin; α2-β2-γ1 chains, laminin 221). The laminin β2 chain has an organizational role at the neuromuscular junction, where it is concentrated ( ). Contrary to common belief, however, appreciable amounts of laminin β2 are detected on the extrajunctional sarcolemma, particularly in mature muscle (see Fig. 6.9e ; ). The difference probably relates to the use of different antibodies in the various studies. The laminin α5 chain is detected on blood vessels and is highly expressed on immature fibres. It is down-regulated during development and only a little is seen on the sarcolemma of mature human muscle fibres (see Fig. 6.9b ). The clinical severity of cases with a defect in the LAMA2 gene that encodes the laminin α2 chain of laminin 211 (merosin) varies from severe to mild and this is usually reflected in the amount of protein that can be detected. Severe cases usually show an absence, or only very slight traces, of laminin α2 on the sarcolemma ( Fig. 6.10a–c ; ). Milder cases show only a reduction of the protein and it is then necessary to assess both N- and C-terminal epitopes of this large protein ( ; see Ch. 12 ). Secondary changes in laminin α2, α5 and β1 chains are discussed below. No defects in laminin γ1 have been identified and it is therefore useful for assessing the integrity of the basal lamina.
Defects in the genes that encode three chains of collagen VI are responsible for Bethlem myopathy and the Ullrich form of congenital muscular dystrophy (see Ch. 12 ; ). Distinct labelling of collagen VI associated with the sarcolemma is seen in normal muscle ( Fig. 6.11 ), and it is also seen in the perimysium and endomysium when there is excess fibrosis. In the milder cases of Bethlem myopathy, many of which are dominantly inherited, an alteration in collagen VI immunolabelling is rarely seen ( Fig. 6.12 ; but see Fig. 12.9 ). In recessive cases of Ullrich congenital muscular dystrophy and cases intermediate between Ullrich congenital muscular dystrophy and Bethlem myopathy, however, collagen VI may be absent or severely reduced (see Fig. 6.12b, c ). In some cases, the reduction may only be a subtle change that is only apparent at the sarcolemma (see Fig. 6.12c, d ). It is important that the good preservation of the basal lamina/basement membrane is controlled with parallel double-labelling studies of another basal lamina/basement membrane protein, such as collagen IV, collagen V or perlecan. Collagen V has a similar localization to collagen VI, and perlecan interacts with collagen VI (see below).
Missense mutations and in-frame insertions in the gene for perlecan have been reported in Schwartz–Jampel syndrome, but the protein is still present ( ). Complete absence of the protein is lethal and occurs in dyssegmental dysplasia with severe lack of bone growth. Perlecan has a major role in clustering of acetylcholine esterase to the neuromuscular junction and connects the basal lamina to the plasma membrane via integrins.
Mutations in others collagen genes, including collagen I, III and V. are associated with Ehlers–Danlos syndrome in which neuromuscular symptoms can be present ( ). Heterozygous mutations in the COL42A gene that encodes the 2A chain of collagen IV have been reported in a few patients with a myopathic phenotype resembling muscle-eye-brain disease/Walker–Warburg syndrome, as well as being associated with other phenotypes ( ). Mutations in collagen IX are associated with epiphysial dysplasia and a myopathy ( ), but immunohistochemical studies of the defective proteins in muscle are limited. Mutations in collagen XII cause a disorder similar to Ullrich CMD and abnormalities in expression of the protein can be detected (see ch.12). An abnormality in the expression of tenascin–X has also been shown in some patients with Ehlers–Danlos syndrome, a disorder that is associated with a variety of genetic defects ( ).
Another primary defect in a membrane-associated protein is integrin α 7 , which interacts with laminin α2 ( ). Defects in the gene cause a mild disorder classified as a congenital muscular dystrophy but it is generally accepted that the features are ‘myopathic’ rather than ‘dystrophic’. Absence of integrin α7 is seen with a concomitant decrease in its partner, integrin β1D. This disorder is very rare, and the original studies were not performed with a commercial antibody (see Ch. 12 ). Integrin α7β1D forms a transmembrane complex that interacts with several proteins, including laminin α2, and secondary alterations in integrins occur (see below).
The lysosomal-associated membrane protein 2 (LAMP-2) is a transmembrane lysosomal protein that is defective in Danon disease (see Ch. 16 ). This X-linked disorder is associated with cardiomyopathy, a vacuolar myopathy and variable degrees of mental retardation ( ). Immunohistochemistry and immunoblots show a virtual absence of LAMP-2 protein ( ), as well as several secondary abnormalities (see Ch. 16 ).
A protein of the endoplasmic reticulum membrane required for the assembly of the vacuolar ATPase complex, encoded by the VMA21 gene, is responsible for the X-linked myopathy with excessive autophagy (XMEA; ). The muscle pathology shares some similarities with Danon disease (see Ch. 16 ), but there are no immunohistochemical studies of the primary gene product.
Primary Defects in Nuclear Proteins
The nuclear envelope is composed of an outer and inner membrane, nuclear pore complexes and a lamina. The outer membrane is continuous with the endoplasmic reticulum, and a number of proteins are integral components of the inner membrane. Several proteins of the nuclear cytoskeleton and nuclear envelope interact and form a complex ( ). Some of these proteins are known to have pathogenic consequences in neuromuscular disorders (emerin, lamin A/C, nesprin 1 and 2, TMEM43), while others (e.g. SUN1 and SUN2) are modifiers (see Ch. 13 ). Emerin is anchored to the inner nuclear membrane ( Fig. 6.13 ), and mutations in the gene are responsible for the X-linked form of Emery–Dreifuss muscular dystrophy (see Ch. 13 ). The majority of mutations in the EMD gene encoding emerin result in truncation of the protein, which lacks the C-terminal domain, and result in a total absence of the protein from all nuclear membranes. This absence is easily detected by immunohistochemistry of muscle, skin or buccal cells ( ). Very rare exceptions to this have been reported, and emerin was seen to be reduced ( ) or mislocalized in the muscle cytoplasm ( ).
Lamins are a principal component of the nuclear lamina, and mutations in the gene for lamin A/C ( LMNA ) are associated with several phenotypes, including the autosomal dominant form of Emery–Dreifuss muscular dystrophy (see Ch. 13 ), dilated cardiomyopathy, limb-girdle muscular dystrophy 1B, familial partial lipodystrophy, an axonal neuropathy (Charcot–Marie–Tooth type 2B1), mandibuloacral disease and, premature ageing disorders ( ). Immunolabelling of lamin A/C in dominant Emery–Dreifuss muscular dystrophy of muscle shows no detectable difference from controls. Secondary changes in laminin β1, however, may be seen (see below).
The nuclear membrane proteins nesprin-1 , nesprin-2 and TMEM43 (encoded by SYNE-1 , SYNE-2 and TMEM43 , respectively) have also been shown to be associated with myopathic disorders (see ). Nesprin-1 has been shown to be associated with a severe recessive disorder with arthrogryposis ( ), and in patients with cardiac conduction defects and an Emery–Dreifuss muscular dystrophy phenotype ( ). An absence of nesprin-1 can be demonstrated in some patients (see Figure 13.10 ). In control muscle labelling of nuclear membrane proteins is similar in peripheral and internal nuclei ( Fig. 6.13 ) except in regenerating fibres labelled with antibodies to nesprin-1 (see Ch.13 ). Heterozygous mutations in TMEM43 have also been identified in two cases with an Emery–Dreifuss muscular dystrophy phenotype ( ). Immunohistochemical studies in these cases have been limited, but fibroblasts and cells expressing the mutant protein have shown reduced or abnormal immunolabelling of nuclear membrane proteins. A muscle biopsy from one of the cases with a TMEM43 mutation was reported to show reduced nuclear labelling of TMEM43 ( ).
Mutations in the gene encoding the nuclear matrix protein matrin-3 cause an autosomal dominant distal myopathy with vocal cord and pharyngeal weakness ( ). Matrin-3 immunohistochemical labelling showed reduced labelling of some nuclei in the only biopsy available for study, but the sample was of end-stage muscle.
Other disorders are also caused by defects in nuclear proteins, but immunohistochemistry currently has a limited role in studies of the primary protein defect. These include the survival motor neurone (SMN) protein in spinal muscular atrophy, the expansion of the genes responsible for both forms of myotonic dystrophy and the expansion of the poly(A)-binding protein nuclear 1 gene ( PABPN1 ) in oculopharyngeal muscular dystrophy (OPMD). Antibodies to PABPN1 have been shown to localize to the nuclear inclusions in patients with OPMD (see Ch. 14 ; ). Antibodies to PABPN1, however, can also label nuclei in controls, and high potassium is needed to show specificity for OPMD (see Ch. 14 ).
Primary Defects in Myofibrillar and Cytoskeletal Proteins
An increasing number of defective genes have been identified in a number of proteins related to myofibrillar proteins. They result in myofibrillar disorganization and, collectively, the term ‘myofibrillar myopathy’ has been used for one of these groups of disorders (see Ch. 16 ). The myofibrillar myopathies highlight the importance of proteins that interact with Z line proteins and maintain sarcomeric structure ( ). Myofibrillar proteins affected by mutations in the corresponding gene include myosin heavy chain isoforms, skeletal actin, telethonin, myotilin, ZASP, Bag3, αB-crystallin, filamin C, FHL1, troponin isoforms, tropomyosin, titin, nebulin and the intermediate filaments desmin and plectin. The gene for the intermediate filament protein syncoilin has also been screened as a possible candidate for a myofibrillar myopathy, but no mutations have been found ( ). These defective proteins can all be detected by immunohistochemistry but as some relate to a dominant condition, and/or the mutations are often missense, a total absence of the protein is rarely seen. Sometimes, however, an accumulation of protein occurs—for example, desmin, myotilin or slow myosin (see below)—and additional secondary changes can be detected (see Ch. 16 ).
Myosin heavy chains are encoded by a multigene family and exist in several isoforms that are regulated in a tissue and developmental specific manner ( ). The physiological fast or slow characteristics of the different fibre types relate to the respective isoforms. The major myosin heavy chain isoform in slow, type 1 fibres is encoded by the MYH7 gene on chromosome 14. This is also the major isoform in cardiac ventricles (β-cardiac myosin heavy chain) and mutations are a cause of familial cardiomyopathy ( ). Mutations in the rod domain of MYH7 have been identified in patients with no overt cardiomyopathy but a skeletal muscle myopathy, sometimes with myosin storage ( ). Immunohistochemistry shows these inclusions are in type 1 fibres and contain slow myosin (see Ch. 15 ). This disorder has been classified as a protein surplus myosin storage myopathy ( ). And mutations in the 3′ domain of MYH7 have been shown to cause a distal myopathy ( ). Thus, mutations in different domains of this gene lead to dominant disorders with varying phenotype. A few patients with recessively inherited mutations in the MYH7 gene have also been reported ( ).
Both dominant and recessive inheritance of mutations in the fast myosin heavy chain IIa gene ( MYH2 ) on chromosome 17 have been found (see Ch. 15 ; ). In addition to the presence of rimmed vacuoles, immunolabelling of fast myosin shows the fast 2A fibres are selectively affected, being atrophic, or absent ( ). Mutations in genes encoding developmental myosins ( MYH8 and MYH3 ) are associated with syndromes that present with distal arthrogryposis (see Ch. 15 ; ), but there is no information on their immunohistochemical localization in muscle samples. Similarly, immunohistochemical studies of myosin-binding protein C in cases with mutations in the corresponding gene are limited ( ).
Mutations in the ACTA1 gene encoding sarcomeric skeletal muscle actin are now known to be associated with a broad spectrum of clinical phenotypes in forms of nemaline myopathy, ranging from severe to mild (see Ch. 15 ; ). Mutations can give rise to actin accumulation, with or without nemaline rods, which may be nuclear and/or cytoplasmic. Mutations in the ACTA1 gene also result in congenital fibre type disproportion without any other structural defect ( ) and in a dominant congenital myopathy characterized by cores devoid of mitochondria and disrupted myofibrils and type 1 fibre predominance, as in central core disease, but no nemaline rods ( ). A mutation in the ACTA1 gene has also been found in the rare case described as ‘zebra body myopathy’, and actin accumulation as well as nemaline rods and zebra bodies were observed ( ).
Immunohistochemical studies of tropomyosin isoforms in human muscle as a primary defect are limited, but mutations in the genes for α-tropomyosin and β-tropomyosin are responsible for rare dominant forms of nemaline myopathy and cap-like structures (see ). Mutations in the gene for β-tropomyosin can also cause distal arthrogryposis without the presence of rods ( ). The primary role of tropomyosin in relation to cardiomyopathies has also been established ( ).
Isoforms of α -actinin have been characterized and mainly studied in relation to fibre typing and the rods in nemaline myopathy, rather than as a primary cause of a muscle disorder. Antibodies to α-actinin-2 label all muscle fibre types, while antibodies to α-actinin-3 only label a subset of fast fibres. A common, non-pathogenic polymorphism has been found in the gene for α-actinin-3 ( ACTN3 ) which results in an absence of α-actinin-3 from all fibres and may have some athletic advantage ( ).
Titin and nebulin are two of the largest proteins known, both of which exist as multiple isoforms resulting from differential splicing ( ). They have a role in maintaining myofibrillar alignment and length and contribute to the elasticity of the myofibrils. The genes for both proteins are located on chromosome 2q, and mutations in the nebulin gene are responsible for one of the more common forms of nemaline myopathy ( ; ). Commercial antibodies to nebulin in patients with mutations in the NEB gene show retention of the protein, but research studies with an antibody corresponding to the SH3 region of nebulin have shown an absence in a few severe cases of nemaline myopathy, which can help direct molecular analysis ( ). Studies have also shown a reduction of nebulin on immunoblots in some severe cases with NEB mutations ( ). Recently, monoclonal antibodies that specifically recognize exon 143 or 144 of nebulin have been raised ( ). These two exons are differentially spliced in human muscle and studies suggest that they are developmentally regulated and that exon 143 is more highly expressed in fibres with fast myosin ( Fig. 6.14 ; ). Titin, encoded by the TTN gene, is involved in neuromuscular disorders with varying phenotype, including limb-girdle dystrophy 2J, dominant hereditary myopathy with early respiratory failure (HMERF), some early-onset cases with a fatal cardiomyopathy and a congenital myopathy as well as cardiomyopathies (see Chapter 11, Chapter 15, Chapter 16 ; ). As with nebulin, absence of the whole titin protein does not occur, but a non-commercial antibody raised against the transcript from the defective last exon of this giant gene has shown an absence in LGMD2J ( ). Secondary abnormalities in nebulin expression occur in DMD and, prior to the identification of the dystrophin gene, nebulin was thought to be the primary defective gene product ( ), but this was subsequently found to be a secondary effect.
Mutations affecting other myofibrillar proteins in which immunohistochemistry has been used to study the primary protein include telethonin , responsible for limb-girdle muscular dystrophy 2G, myotilin , responsible for limb-girdle muscular dystrophy 1A and a myofibrillar myopathy, and slow troponin T , responsible for a rare form of nemaline myopathy ( ). The prevalence of these defects is not known, as only a few affected patients have been reported. Limb-girdle muscular dystrophy 2G was thought to be confined to the Brazilian population, but rare cases elsewhere in the world have been identified which show an absence of telethonin protein with immunolabelling ( ) or, occasionally, retention of telethonin ( ). Studies of cases with mutations in the gene for myotilin suggest they may be a common cause of myofibrillar myopathy in some populations (see Ch. 16 ; ). Mutations in the gene for a fast isoform of troponin I on chromosome 11 cause a form of distal arthrogryposis ( ), but there are currently no immunohistochemical data on the expression of the protein although abnormalities have been detected in immunoblots ( ).
The cytoskeletal protein desmin is involved both primarily and secondarily in myofibrillar myopathies often collectively referred to as ‘desminopathies’, ‘desmin-related myopathies’ or ‘myofibrillar myopathies’ (see Ch. 16 ; ). Both missense mutations and deletions in the gene for desmin have been identified ( ). Inheritance is often dominant but rare recessive cases are also known. Age of onset is variable, and cardiomyopathy is a frequent complication. Antibodies to desmin show accumulation in fibres that do not express fetal myosin, distinguishing them from regenerating fibres with high desmin. No desmin was detected in the muscle by immunohistochemistry and immunoblotting from two siblings heterozygous for null mutations in the DES gene ( ). A characteristic accumulation of electron-dense granulofilamentous material is seen at the ultrastructural level (see Ch. 5 ), and additional features include secondary accumulation of a variety of proteins and cytoplasmic and spheroid bodies (see Ch. 16 ). Similar pathological features are also seen when other genes in this group of disorders causing a myofibrillar myopathy are affected ( ). The gene encoding plectin is mutated in the rare skin blistering recessive disorder, epidermolysis bullosa simplex with muscular dystrophy, and is associated with a range of neuromuscular phenotypes ( ). The blistering may not always be marked ( ), and the gene can also be associated with a myasthenic syndrome (see Ch. 21 ; ) and a form of limb-girdle muscular dystrophy (see Ch. 11 ; ). Plectin has a role in mitochondrial function, in organizing the intermediate filament cytoarchitecture, including anchoring desmin to the nuclear membrane, to the periphery of Z lines and at the sarcolemmal costameres ( ). Immunolabelling of skin and muscle with antibodies to plectin shows an absence or reduction and that a secondary accumulation of desmin can occur in epidermolysis bullosa simplex ( ). Plectin also interacts with β-dystroglycan, dystrophin and utrophin ( ) and secondary abnormalities occur in other disorders (see below). Alternative splicing of plectin transcripts gives rise to eight protein isoforms, of which four are highly expressed in muscle (1, 1b, 1d and 1f). Mutations affecting the 1f isoform have been found in a recessively inherited form of limb-girdle muscular dystrophy, and a reduction in sarcolemmal plectin immunolabelling was reported, and also of the corresponding RNA ( ). Internal labelling of plectin was retained in muscle fibres, and studies of mouse muscle, using isoform-specific antibodies, have shown that this internal labelling is higher in 2A fibres ( ). This publication also showed that the plectin 1d isoform is localized to the Z line. We are not aware of studies with isoform-specific antibodies in diseased human muscle, and those published have been with antibodies that recognize all isoforms, but alterations in plectin localization can be observed with them.
Primary Defects in Enzymes
Several primary enzyme defects are known to cause a neuromuscular disorder, but it is often more important to examine the activity of the enzyme biochemically rather than its localization by immunohistochemistry. Immunoblots, however, are useful for studying enzymes such as calpain-3 , which is defective in limb-girdle muscular dystrophy 2A ( ). This disorder is unusual among the limb-girdle muscular dystrophies in not being caused by a membrane-associated protein. Calpain-3 is an enzyme located in the cytosol of the fibre and in the nucleus, and it binds to the C-terminus of titin. Absence of calpain-3 can be detected on sections but a reduction is more difficult to assess and requires immunoblots when secondary changes affecting dysferlin and caveolin-3 can also be assessed ( ).
The valosin-containing protein (VCP, also known as p97) and UDP-N-acetylglucosamine 2-epimerase/N-acetylmannosamine kinase (GNE) are two enzymes associated with myopathies often characterized by vacuoles (see Ch. 16 ). Mutations in the VCP gene cause inclusion body myopathy with Paget bone disease and frontotemporal dementia (IBMPFD) and the phenotype now also includes amyotrophic lateral sclerosis (ALS), Parkinson disease, cardiomyopathy and Charcot–Marie–Tooth disease ( ). VCP is a ubiquitously expressed triple A ATPase that has an important role in the ubiquitin proteosome protein degradation system and aggresome formation, as well as other cell functions that include the cell cycle, nuclear envelope reconstruction, postmitotic Golgi reassembly, DNA damage response and suppression of apoptosis ( ). Immunohistochemistry of muscle biopsies shows accumulation of nuclear and sarcoplasmic VCP and TDP-43. MHC I is sometimes up-regulated and sometimes inflammatory cells are present ( ), but these are not consistent features. Lobulated fibres, ring fibres and some polyglucosan-like bodies have also been reported in a patient with a mutation in the VCP gene who had asymmetrical scapuloperoneal weakness and head drop without Paget disease or dementia ( ).
The myopathy caused by mutations in the gene encoding GNE (also known as Nonaka myopathy, distal myopathy with rimmed vacuoles or hereditary inclusion body myopathy) is a distal myopathy that spares the quadriceps, a feature that led to the disease also being called ‘quadriceps-sparing myopathy’ (see Ch. 16 ; ). GNE is an enzyme involved in the biosynthesis pathway of sialic acids and the reduced enzyme activity of GNE is thought to involve hyposialylation of glycoproteins, although this does not explain all of the pathogenic mechanism ( ). A variety of research studies have examined sialylation of various proteins in GNE myopathy that include β1-integrin, NCAM, α-dystroglycan, although the latter is not considered to have a major role in GNE myopathy ( ).
Immunohistochemical studies of primary defects in mitochondrial enzymes are increasing. Abnormalities in mitochondrial oxidative phosphorylation are associated with several multisystem disorders (see Ch. 18 ). The traditional method to study this in muscle biopsies has been histochemical analysis of succinic dehydrogenase and cytochrome c oxidase (see Chapter 18, Chapter 3, Chapter 4 ) which localize the activity of these mitochondrial specific enzymes ( ). Antibodies to complex I–IV, however, are proving useful in some conditions but do not detect defects in all mitochondrial-related disorders ( ). Complex I deficiency can be identified by antibodies to this component (see Ch. 18 ), and a quadruple multiplex immunofluorescent method has been developed to examine complex I, complex IV and mitochondrial mass within fibres labelled for laminin ( ). Antibodies to TOMM22 and porin are useful for labelling all mitochondria ( ). Antibodies to mitochondria show a fibre-typing pattern comparable to staining to cytochrome c oxidase but are particularly useful in identifying a deficiency of complex I (see Ch. 18 ).
Primary Defects of Ion Channels
The main ion channels that have been studied by immunohistochemistry are the ryanodine receptor 1 (RYR1), the dihydropyridine receptor (DHPR) and the sarcoendoplasmic reticulum calcium ATPase (SERCA) (see Ch. 20 ). Mutations in the RYR1 gene on chromosome 19q occur in core myopathies and malignant hyperthermia (see Ch. 15 ; ). Malignant hyperthermia susceptibility is also linked to several other loci and genes (see Ch. 20 ; ). Not all cases of RYR1 -related core disease appear to be susceptible to malignant hyperthermia but patients are always advised of the potential risk. The ryanodine receptor is a large ligand-gated calcium-release channel with an essential role in excitation–contraction coupling ( ). It has several C-terminal transmembrane loops in the membrane of the sarcoplasmic reticulum and cytoplasmic regions that interact with the voltage-gated dihydropyridine receptor of the T tubule. Genotype–phenotype correlations suggest that mutations in the transmembrane C-terminal region of RYR1 are more commonly, but not exclusively, associated with central core disease, whereas malignant hyperthermia is more often associated with N-terminal hot spots ( ). Many mutations in the RYR1 gene are missense and dominantly inherited, so total absence of the protein is not seen, nor in the patients with recessively inherited mutations. Limited immunohistochemical studies of the RyR1 protein have shown a reduction in some cases but they are not currently used for diagnosis. A secondary accumulation of several proteins, however, can be useful for visualizing the cores (see below).
Brody disease, characterized by stiffness and exercise-induced pain ( ), is caused by mutations in the ATP2A1 gene encoding the sarcoplasmic reticulum calcium ATPase, SERCA1 ( ). SERCA1 is confined to fast fibres and SERCA2 to slow fibres. Thus, antibodies to these show a checkerboard pattern in normal muscle. In Brody disease an absence of SERCA1 from the fast fibres can be detected. Proteins, such as sarcolipin, that are associated with SERCA are candidates for Brody syndrome, which clinically resembles Brody disease, but no mutations have yet been found ( ).
Secondary Defects
A large number of publications describe the wide variety of secondary alterations in protein localization and expression that occur in diseased muscle as a consequence of a primary defect in another gene, such as those described previously. In this section we concentrate on those that we find to be of diagnostic value and situations where antibodies can clarify the nature of a structure ( Table 6.3 ). Further details are also discussed in chapters related to particular disorders.
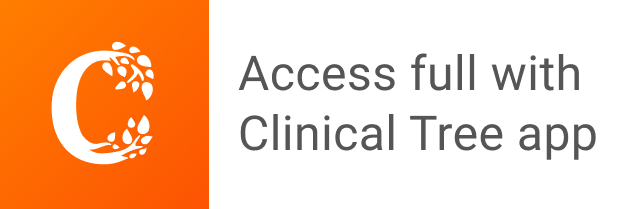