Imaging of the Spine
Amit Jain
Jay A. Khanna
Uma Srikumaran
Introduction
Along with a comprehensive history and physical examination, imaging is an important component of the evaluation of patients with known or suspected spinal pathology. Imaging modalities include conventional radiography, computed tomography, fluoroscopy, myelography, magnetic resonance imaging, and nuclear scintigraphy. An understanding of the differences in the various available modalities and their appropriate use is crucial to guide decisions about patient care. The goals of this chapter are to provide an overview of the various commonly used imaging modalities and to discuss the benefits of each in a variety of clinical scenarios.
Overview of Modalities
Conventional Radiography
Since Wilhelm Roentgen’s discovery of x-rays in 1895, the use of conventional radiography for medical applications has been widespread. Conventional radiography relies on the principle that ionizing radiation is transmitted through tissue differentially on the basis of the tissue’s properties. The ionizing radiation that is used is on the x-ray band of the electromagnetic spectrum. Tissues such as bone, which are rich in high–atomic-weight calcium, absorb x-ray radiation relatively efficiently and reduce the amount of radiation that is transmitted on to the detector, creating a high signal. Soft tissues and intervertebral disks, which contain relatively more water, attenuate x-rays less and, thus, create a low signal on the detector.
Traditionally, x-ray detectors used photosensitive materials such as silver halide, which sustains a photochemical change in response to ionization radiation. Exposed areas, such as those through soft tissue, result in darkening of the crystals, and thus creation of contrast. Modern x-ray detectors are frequently semiconductor-based and can be used for acquisition of digital images.
Conventional radiography is an excellent choice for initial evaluation of the spine. Evaluation typically begins with anteroposterior (AP) and lateral radiographs and can be augmented with use of oblique, flexion-extension, and specific views such as a swimmer’s view of the cervical spine. Radiographs can be obtained quickly and cost-effectively for a variety of scenarios including:
evaluation of trauma
quantification of coronal and sagittal plane deformities
identification of spondylosis, spondylolysis, and spondylolisthesis (Fig. 2.1)
detection of bony lesions such as primary neoplasms and metastatic disease
Further, because of the ease of obtaining them and the relatively low exposure of patients to radiation, conventional radiographs are the first-line imaging modalities for preoperative evaluation, intraoperative instrumentation placement, and postoperative follow-up.
Although conventional radiographs can be useful in understanding bony alignment and anatomy, they are limited in their ability to show soft tissue components of the spine such as intervertebral disks, spinal cord, nerve roots, and bone marrow quality. Generally, more than 30% to 50% loss in trabecular bony architecture is required to show a reduction in bony mass on radiographs. Nonetheless, understanding the use of conventional radiography and its appropriate interpretation is paramount for the practitioner who wishes to evaluate the spine.
Computed Tomography
Since its introduction in the 1970s, computed tomography (CT) has become a vital tool for evaluation of a variety of medical conditions. The use of CT scans in the spine is widespread for understanding the bony anatomy and evaluating a variety of pathologies.
CT technology relies on obtaining numerous x-rays, simultaneously captured on a circumferential detector. Modern CT detectors rely on photodiodes and modern scintillation materials that can capture a large amount of x-ray data in a very short period of time. The output of a CT scan is projection data, known as Radon transform, which is mathematically inverted using computational methods to reconstruct the original density data. Mean attenuation of the x-rays that pass through a tissue are represented in a CT scan as pixels on the Hounsfield scale. When thickness of the slice is taken into account,
the resulting unit is a voxel. The Hounsfield scale is a linear transformation of the original linear attenuation data into one that uses radiodensity of distilled water as a reference of 0 Hounsfield units (HU). The range of HU varies depending on the tissue, with bone generally between 700 and 3,000 HU, cerebrospinal fluid approximately 15 HU, and blood 30 to 45 HU.
the resulting unit is a voxel. The Hounsfield scale is a linear transformation of the original linear attenuation data into one that uses radiodensity of distilled water as a reference of 0 Hounsfield units (HU). The range of HU varies depending on the tissue, with bone generally between 700 and 3,000 HU, cerebrospinal fluid approximately 15 HU, and blood 30 to 45 HU.
The large amount of data obtained via a CT scan can be used to provide fine spatial anatomic details, which cannot be obtained using conventional radiography. Post-image acquisition algorithms are frequently used to construct axial, sagittal, and coronal reconstructions. The voxel data can also be used to create three-dimensional reconstructions. Data acquisition is generally rapid and yields excellent visualization of anatomic details. A recent meta-analysis concluded that CT had significantly greater sensitivity and outperformed plain radiography as a screening test for patients at very high risk of cervical spine injury. Thus, CT should be preferred over plain radiography in unconscious patients.
CT is particularly useful for characterizing bony abnormalities in regions where conventional radiography cannot be used such as the occipitocervical junction (Fig. 2.2), sacrum, and coccyx. The ability to create axial plane views and three-dimensional reconstructions can enhance the surgeon’s ability to visualize complex anatomic regions (Fig. 2.3) for evaluation of complex deformities, fractures (Fig. 2.4), and neoplasms. Further, certain types of procedures such as myelography (section below) and biopsy frequently rely on CT guidance. Newer, imaging-guided surgical navigation systems also use CT-generated data.
Despite its many benefits, CT has some drawbacks, including poor soft tissue contrast (compared with MRI), exposure to more ionizing radiation (compared with conventional radiography), and sensitivity to motion and metal artifacts. Newer-generation CT algorithms are being developed to minimize the effects of motion and metal artifacts. Because of the potential risk of ionizing radiation to the fetus, CT scans are generally avoided in pregnant women.
Fluoroscopy
Conventional fluoroscopy relies on the use of continuous low-energy x-rays, which are passed through the region of interest to provide a real-time, two-dimensional image of the anatomy. The real-time and potentially continuous nature of the visualization allows fluoroscopy to be used for a variety of purposes, including
dynamic evaluation of spinal pathology, interventional procedures such as facet joint and epidural injections, and intraoperative placement of spinal instrumentation. Fluoroscopy is crucial for minimally invasive spine surgery, where dissection is limited, and instrumentation is placed percutaneously. Fluoroscopic equipment is available in many configurations suited to different clinical applications. Mobile C-arms are generally used for intraoperative spinal instrumentation placement and confirmation.
dynamic evaluation of spinal pathology, interventional procedures such as facet joint and epidural injections, and intraoperative placement of spinal instrumentation. Fluoroscopy is crucial for minimally invasive spine surgery, where dissection is limited, and instrumentation is placed percutaneously. Fluoroscopic equipment is available in many configurations suited to different clinical applications. Mobile C-arms are generally used for intraoperative spinal instrumentation placement and confirmation.
Although the radiation exposure to the patient from fluoroscopic imaging is generally episodic and limited, frequent use of fluoroscopy may lead to radiation exposures to the surgeon or health care provider that are beyond maximum recommended limits. Furthermore, although the fluoroscopy apparatus uses low-energy x-rays, the total radiation exposure with fluoroscopy can frequently exceed that of conventional radiography. As with the use of any ionizing radiation imaging modality, personal protective equipment should be worn.
Myelography
Myelography consists of injection of contrast medium into the spinal canal to detect pathology of the spinal cord, nerve roots, and any occupying lesions in real time. It is often used in conjunction with fluoroscopy or CT and involves injection of a radiopaque dye, which usually consists of nonionic, water-soluble agents such as iohexol or metrizamide. Ionic, water-soluble media such as iothalamate meglumine have adverse risk profiles and are unsuitable for administration during myelography. Myelography involves injection of the contrast material into the subarachnoid space using lumbar or cervical puncture. Once the contrast material has distributed through the subarachnoid space, a conventional high-resolution CT scan is often obtained.
The widespread use of multi-planar, high-resolution CT scanners and advanced magnetic resonance imaging (MRI) techniques has resulted in a decline in the use of myelography for evaluation of the spine recent years. Nonetheless, several indications for myelography exist, including for patients in whom MRI is contraindicated because of the presence of a pacemaker or defibrillator, or in patients with severe claustrophobia. The images obtained from myelography can be used to assess compression on the spinal cord or nerve roots, especially stenosis adjacent to spinal instrumentation or
other metallic artifact (Fig. 2.5), where MRI may produce imaging artifacts that preclude the evaluation, for example, of foraminal stenosis.
other metallic artifact (Fig. 2.5), where MRI may produce imaging artifacts that preclude the evaluation, for example, of foraminal stenosis.
The disadvantages of myelography include the invasive nature of the procedure, which carries a risk of infection and meningitis, potential allergic reaction to the contrast material, and frequent post-procedure headaches and nausea. Further, myelography should be avoided in patients with seizures or bleeding disorders and in those taking anticoagulation medication. Myelography uses ionizing radiation and carries the risks of radiation.
Magnetic Resonance Imaging
Since its introduction in 1971 by Paul Lauterbur, MRI has proved to be an invaluable tool for the evaluation of the spine because of its ability to demonstrate and differentiate soft tissues on the basis of their composition and tissue type. MRI relies on the fact that different tissues have different rates of return to equilibrium after excitation on the basis of the content of water molecules when subject to oscillating radiofrequency signals applied under a strong, uniform magnetic field. Differences in proton density in the body can be converted to high-resolution images. Tissues such as muscle and ligaments, which have a high quantity of protons, exhibit a high degree of attenuation under the magnetic field, and yield high-intensity signals on MRI. Tissues such as periosteum and cortical bone are generally low in water content and yield low-intensity signals on MRI.
The appearance of magnetic resonance images varies on the basis of the type of pulse sequence used. With traditional spin-echo imaging, a radiofrequency pulse is applied, causing protons in the water molecules, which are aligned parallel to the external magnetic field, to flip by 90 degrees. When the stimulant pulse is removed, the protons realign themselves parallel to the external magnetic field. The time it takes for 63% of the nuclei to realign themselves with the external field after the radiofrequency terminates is the T1 relaxation time. The T1 time varies with the proton content of the tissue. For example, the T1 time of fat is shorter than that of water because the latter has a higher proton density.
T1-weighted images are generally used for the evaluation of anatomic detail. Air, soft tissue, and fast-moving blood generally appear as very low signal intensity or dark. Tissues with high water content such as cerebrospinal fluid, cartilage, and muscles also manifest as low signal intensity. On T1-weighted images, high-intensity signals are yielded from tissues with low water content, such as fat, fatty bone marrow, slow-flowing blood, and contrast agents.
T2 decay time refers to the loss of transverse plane magnetization after the radiofrequency pulse is terminated. Proton-poor tissues such as fat have faster T2 decay than water because they contain fewer protons to maintain magnetization in the transverse plane. T2-weighted images are generally used for identification of pathology because diseased tissue often has high water content. In T2-imaging, fat, bone marrow, and muscles generally have low- to intermediate-intensity signals, and cerebrospinal fluid and cartilage have high-intensity signals. In both T1-weighted and T2-weighted imaging,
cortical bone, tendons, and ligaments generally yield low- to intermediate-intensity signals. Advanced techniques such as short tau inversion recovery (STIR) and fluid-attenuated inversion recovery (FLAIR) are frequently used for differential suppression of the fat and water signals, respectively.
cortical bone, tendons, and ligaments generally yield low- to intermediate-intensity signals. Advanced techniques such as short tau inversion recovery (STIR) and fluid-attenuated inversion recovery (FLAIR) are frequently used for differential suppression of the fat and water signals, respectively.
The MRI scan may be enhanced by the use of paramagnetic contrast agents, most frequently gadolinium- based agents. Contrast agents can help in evaluation of degenerative (Fig. 2.6), inflammatory, and neoplastic processes, as well as in identification of vascular lesions and revascularization. Contrast agents are generally
used in the setting of T1-weighted image acquisition and frequently shorten the T1 relaxation of nearby protons, thus enhancing image contrast. The recommended intravenous dose of contrast agent is 0.1 mmol/kg of body weight. Gadolinium is renally cleared and should be avoided in patients with kidney disease to avoid risk of nephrogenic systemic fibrosis. Advantages of MRI include the lack of ionizing radiation and the ability to produce high-resolution images for a variety of disease processes such as infection, spinal neoplasms, degeneration, stenosis, and cord pathologies. Disadvantages include long data acquisition time (compared with CT), use of magnetic fields (which precludes patients with embedded metallic bodies such as intracranial clips, intraocular implants, and pacemakers), image artifacts in the setting of spinal instrumentation, and relative costliness (compared with conventional radiography and CT). Although MRI does not involve exposure to ionizing radiation, as in the case of CT and myelography, the potential risks of exposure to the magnetic field during pregnancy are not well established.
used in the setting of T1-weighted image acquisition and frequently shorten the T1 relaxation of nearby protons, thus enhancing image contrast. The recommended intravenous dose of contrast agent is 0.1 mmol/kg of body weight. Gadolinium is renally cleared and should be avoided in patients with kidney disease to avoid risk of nephrogenic systemic fibrosis. Advantages of MRI include the lack of ionizing radiation and the ability to produce high-resolution images for a variety of disease processes such as infection, spinal neoplasms, degeneration, stenosis, and cord pathologies. Disadvantages include long data acquisition time (compared with CT), use of magnetic fields (which precludes patients with embedded metallic bodies such as intracranial clips, intraocular implants, and pacemakers), image artifacts in the setting of spinal instrumentation, and relative costliness (compared with conventional radiography and CT). Although MRI does not involve exposure to ionizing radiation, as in the case of CT and myelography, the potential risks of exposure to the magnetic field during pregnancy are not well established.
Nuclear Scintigraphy
Nuclear scintigraphy, commonly called “radionuclide bone scan,” is an imaging modality that uses radiopharmaceuticals administered intravenously or orally. The radiation emitted from the agents is captured by external detectors, often gamma cameras. Similar to the techniques used in CT scan, the captured radiation is used to create two- and three-dimensional reconstructions of the anatomy. The most commonly used agent for scintigraphy is technetium-99m-labeled phosphate, which emits gamma rays at 140 keV and is readily taken up by osteoblasts. The technique is thus sensitive to situations in which osteoblastic activity is increased such as in cases of metabolic bone disease, stress fractures, and bony reaction to neoplastic (Fig. 2.7) and infectious processes.
A major benefit of nuclear scintigraphy is its ability to detect active osseous remodeling in the entire skeleton in one study. This makes it an ideal modality for performing a skeletal survey and for evaluation of lesions such as spondylolysis, which are associated with substantial bony remodeling. Further, nuclear scintigraphy is more sensitive than conventional radiography for detection of metabolic processes such as osteomalacia, primary hyperparathyroidism, and renal osteodystrophy. However, certain blood dyscrasias such as multiple myeloma and lymphoma, which do not lead to osteoblastic activation, can be missed with a radionuclide bone scan. When suspicion is high for those entities, a skeletal survey with conventional radiography is recommended.
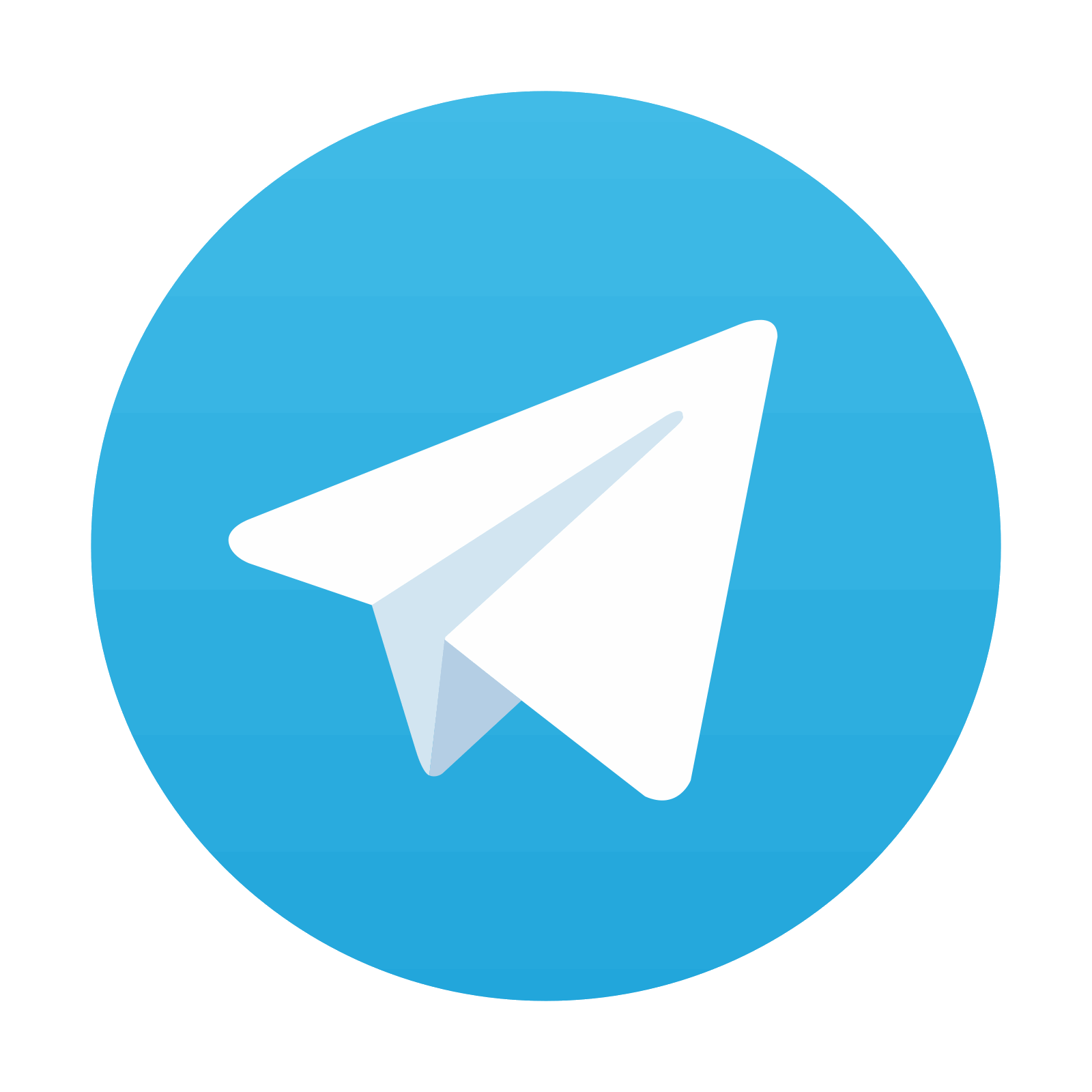
Stay updated, free articles. Join our Telegram channel
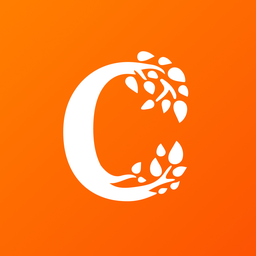
Full access? Get Clinical Tree
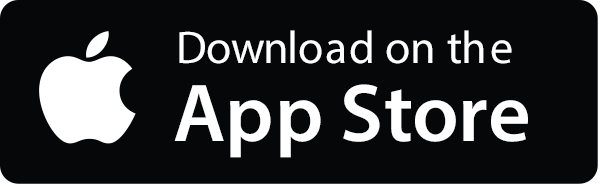
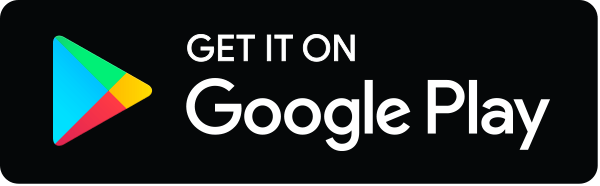