CHAPTER OBJECTIVES
At the conclusion of this chapter, the learner will be able to:
Relate the physiologic effect of hyperbaric oxygen therapy to wound healing.
Identify the diagnoses for which hyberbaric oxygen therapy is indicated.
Recognize the different types of chambers used for hyperbaric oxygen therapy.
Discuss the gas laws as they relate to the physics of pressurization.
State the contraindications for hyperbaric oxygen therapy.
Recognize the possible adverse effects of hyperbaric oxygen therapy.
Hyperbaric oxygen therapy, in its simplest terms, means oxygen under pressure. Hyperbaric treatment pressures are compared to atmospheric or sea-level pressure. This chapter includes the history of air and oxygen under pressure, the physics and physiology of hyperbaric oxygen therapy (HBO2), and the current uses of HBO2 as it applies to wound healing. HBO2, like any other treatment modality, has appropriate indications for its use that are supported by scientific evidence. Unfortunately, it is also used inappropriately by unscrupulous purveyors who use and sell HBO2 for profit in the treatment of disorders for which there is no scientific basis for supporting efficacy. Currently the Undersea and Hyperbaric Medical Society has approved 14 indications for which there is adequate scientific basis for treatment with HBO2 (FIGURE 18-1). Responsible users and providers of HBO2 must remain true to scientific roots while being ever-watchful for opportunities for scientific research into potentially new clinical uses for this remarkable treatment tool.
Hyperbaric therapy has been employed for hundreds of years for a multitude of purposes. The earliest reported uses of hyperbaric treatment date back to the 1600s. A British clergyman named Henshaw completed work that marked the beginning of the compressed-air era during which all hyperbaric treatments were performed only with compressed air, as oxygen was not discovered until 1775 by Priestley. In 1662, Henshaw built a sealed chamber that he called a “Domicilium.”1 Valved bellows controlled chamber pressures, which could be raised or lowered by the adjustment of the valve system. Henshaw believed that acute disease processes of all kinds would respond favorably to increased air pressure, while more chronic diseases would respond better to low pressure. If during the course of treatment, a disease was felt to have become chronic, Henshaw readjusted the valves to create a slight vacuum. Given the small pressure changes possible with such an apparatus, any perceived effects on those patients treated were most certainly based on psychology and not physiology.
In 1879, French surgeon JA Fontaine constructed a mobile operating room on wheels that could be pressurized.2 Many surgical procedures were performed in this unit using nitrous oxide as the anesthetic agent. Due to the increased effective concentration of the nitrous oxide and the increased partial pressure of oxygen at pressure, deeper and arguably safer surgical anesthesia was possible. According to the gas laws, compressed air at 2 atm (defined as pressure two times normal sea level air pressure) yields an effective oxygen percentage of 42% compared to 21% at 1 atm or sea-level pressures. In these surgical procedures, interesting physiologic and anatomic effects were observed. Patients did not have the usual cyanotic color while coming out of anesthesia and hernias were reduced more easily. Fontaine’s experiments with hyperbaric surgery were the only semiscientific efforts during the entire compressed-air era.
The end of the compressed-air era was marked by Orville J. Cunningham, a professor of anesthesia at the University of Kansas in Kansas City.3 He noted, appropriately, that people with heart disease and other related disorders did poorly while living at altitude but improved upon return to sea level. With this concept in mind Cunningham reasoned that exposing people to increased atmospheric pressures would be even more beneficial. During the flu epidemic of 1918, he placed a critically ill and hypoxic resident physician in a chamber that had been designed for animal studies. By compressing the chamber to 2 atm, he was able to keep the patient oxygenated through the hypoxic phase of his illness. Subsequently, Dr. Cunningham constructed a chamber 88 feet long and 10 feet in diameter and began to treat a multitude of diseases, most without any scientific evidence that HBO2 would be an effective treatment.
In the 1920s, Mr Timken of the Timken Roller Bearing Company had a spontaneous recovery from a uremic illness while in Dr Cunningham’s chamber. In gratitude for his newly recovered health, Mr. Timken built for Dr. Cunningham the largest hyperbaric chamber ever constructed (FIGURE 18-2). It was a steel sphere, six stories high and 64 feet in diameter, featuring a dining room, individual patient rooms, and most interestingly, a smoking lounge on the top floor. It was capable of reaching 3 atm pressure.
Cunningham believed there was an organism, unable to be cultured, that was responsible for a myriad of diseases including hypertension, uremia, diabetes, and cancer, and that treatment with increased atmospheric pressure helped control or inhibit this organism. Unfortunately, Dr Cunningham was unable to provide any scientific evidence to support his treatments, and the Cleveland Medical Society, along with the American Medical Association (AMA), ultimately forced him to close in 1930.4
The use of hyperbaric oxygen therapy in clinical medicine began in 1955 with the work of I. Churchill-Davidson. He used increased oxygen environments to augment the effects of radiation therapy in cancer patients.5 Also in 1955, Ite Boerema, Professor of Surgery at the University of Amsterdam in Holland, introduced the use of HBO2 to improve outcomes in cardiac surgery patients. His initial work involved animal experiments using a chamber borrowed from the Royal Dutch Navy. These experiments yielded such promising results that Boerema had a large chamber built as an operating room at the University of Amsterdam, where he conducted surgery under pressure for a variety of cardiopulmonary conditions. The first publication of his work appeared in 1956.6
During this same time period, W.H. Brummelkamp, also of the University of Amsterdam, found that hyperbaric oxygen inhibited the growth of certain anaerobic organisms, most importantly Clostridium perfringens, and his group published its first paper on the hyperbaric treatment of clostridial gas gangrene in 1961.7 In 1962, George Smith and G.R. Sharp at the Western Infirmary in Glasgow, Scotland, studied the treatment of carbon monoxide poisoning.8 Based on early favorable results from their work, a clinical chamber was constructed at Duke University and another built later that same year at Mt. Sinai in New York City. Within a short period of time, similar chambers were constructed in large hospitals coast to coast.
Since the 1960s, HBO2 therapy has seen tremendous growth, but also problems and criticism over utilization and regulatory issues. Based on the promising results seen for gas gangrene, cardiac surgery, and carbon monoxide poisoning, the search for new indications burgeoned. Patients suffering from a myriad of acute and chronic conditions were treated in chambers in the hope that hyperbaric oxygen would provide benefit in conditions for which conventional therapies had failed. Unfortunately these efforts produced more anecdotal reports of positive outcomes than they did scientific data supporting those outcomes. In addition, during this period there was a cadre of HBO2 fanatics who treated a multitude of disorders with no regard for science. Nevertheless, improvements in heart–lung machines and the use of hypothermia led to improved patient outcomes in cardiac surgery and essentially obviated the need for surgical hyperbaric units. As a result, by the early 1970s many of the earlier chambers and programs had closed.
By the mid-1970s it had become clear that there was a very real need for structured and evidence-based guidelines for the use of HBO2 therapy. Only one general textbook on hyperbarics, written by Eric Kindwall of St Luke’s Hospital in Milwaukee in 1970, had been published. Dr. Kindwall remained a leader in the field of hyperbaric oxygen therapy and went on to author and edit three seminal volumes of the highly regarded text Hyperbaric Medicine Practice, which is in its fourth edition.9
The Undersea Medical Society (UMS) was founded in 1967 by a group of U.S. Navy Diving and Submarine Medical Officers, and was conceived as an organization dedicated to diving and undersea medicine. In 1976, an 18-member ad hoc Committee on Hyperbaric Oxygenation was formed, with Eric Kindwall as chairman, to review the field of HBO2 therapy.10 In 1986 at the UMS annual meeting in Japan, the membership voted to change the name of the Undersea Medical Society to the Undersea and Hyperbaric Medical Society (UHMS), an organization encompassing the fields of clinical as well as diving medicine that remains active.
The first “Report of the Committee on Hyperbaric Oxygenation” was published in 1977 and divided disorders treated with hyperbaric oxygen into four categories.6 Category one contained disorders for which there was no question of the efficacy of HBO2 and progressed in degrees of supporting evidence to category four, which comprised disorders for which there was no supporting evidence whatsoever. This report has been updated periodically. The most recent edition was published in 2008 and contains the 14 disorders presently approved for treatment with HBO2 therapy (FIGURE 18-1).11
Definition: Hyperbaric oxygen therapy is a treatment during which a patient inside a chamber breathes 100% oxygen intermittently, at a pressure higher than sea-level pressure.11
While any pressure above 1 atm absolute (sea-level pressure) can technically be considered hyperbaric, it is generally accepted that the pressure must be greater than 1.4 atm absolute (ATA) to be considered hyperbaric therapy. Most clinical treatment protocols call for ambient pressures between 2 and 3 ATA.
HBO2 must be delivered systemically, with the patient in a chamber breathing elevated partial pressures of oxygen through an intact cardiopulmonary system. Topical high-pressure oxygen and containers enclosing individual limbs are not considered HBO2 therapy and there are no legitimate data to support their use.
Using the above definitions and guidelines, HBO2 therapy may be administered in either a monoplace chamber (able to treat one patient at a time) or a multiplace chamber (able to treat as many as 20 or more patients simultaneously). The physiology and partial pressures of oxygen are identical in either of the chamber types, but the manner in which the treatments are carried out varies significantly.
The monoplace chamber is a free-standing unit, typically with steel end caps and a thick, cylindrical acrylic hull (FIGURE 18-3A, B). There are variations on this design in terms of materials and configuration and some are designed to accommodate an inside attendant, although still a monoplace chamber with regard to patient treatment (FIGURE 18-4). Patients are treated in a supine or slightly inclined position on an adjustable bed, which slides into the chamber from a matted gurney on wheels. Depending on the age and sophistication of the chamber, pressures may be set and treatments run either manually or automatically by built-in computer systems. In some of these chambers, the monoplace chamber is compressed with 100% oxygen, in contrast to the multiplace chamber, which is compressed with medical-grade air. It is possible to administer intravenous fluids, monitor blood pressures, and even mechanically ventilate an intubated patient within the monoplace chamber. Dick Clarke and his group at Columbia Richland Medical Center in Columbia, SC, have been leaders in the development of critical care procedures in the monoplace environment and in the education of others in this field.
Multiplace chambers can be cylindrical, spherical, or rectangular in shape and vary greatly in their dimensions, from small two-person chambers all the way to the modern rectangular chambers that can seat 20–30 patients simultaneously (FIGURE 18-5). Patients are typically seated in special seats along the walls of the chamber. Due to volume, these chambers are compressed with medical-grade air rather than with oxygen as is the case with the monoplace chamber. One hundred percent oxygen is then administered to the patients through built-in breathing systems using either an air mask or an over-the-head hood (FIGURES 18-6 and 18-7). These consist of an oxygen-supply hose connected to either a mask or acrylic hood. A multiplace chamber is either single lock in configuration, with a single entrance to a single chamber room, or multilock, in which the chamber has two or three small chambers within it, each able to be pressurized independently, in order to facilitate the ingress and egress of patients, personnel, and supplies. An additional advantage of multiplace chambers is the presence of medical personnel within the chamber during each treatment, allowing hands-on or emergency care if necessary in addition to the ability to “lock-in” or “lock-out” extra personnel, equipment, and supplies.
FIGURE 18-5
Multiplace chamber Outside view of a multiplace chamber that can accommodate up to 30 patients who sit in medical grade air and breathe 100% oxygen through masks or hoods. These chambers require a certified attendant to be inside the chamber during treatment. (Used with permission from Marc R. Kaiser, Boca Raton, FL.)

Experimental chambers come in a wide variety of types and configurations, depending on their intended purpose. FIGURES 18-8 and 18-9 illustrate a large double-lock chamber, and FIGURE 18-10 illustrates a collapsible and portable chamber designed to be carried aboard a ship or dive boat.
The principles of pressure and the behavior of gases under pressure are straightforward and governed by basic and well-accepted physical laws. While other gas laws come into play in HBO2 therapy, understanding several of the most important principles is essential to understanding the effects of HBO2 therapy.
Boyle’s Law states that for a body of gas at constant temperature, the volume of that body of gas is inversely proportional to the pressure. Essentially, as pressure increases, gas volume decreases (FIGURE 18-11). Boyle’s Law describes the behavior of gas bubbles and air within a sealed space.
Charles’ Law states that for a body of gas at constant pressure, the volume is directly proportional to the absolute temperature (FIGURE 18-12). In the clinical hyperbaric environment, it is the inverse of this that can become a concern; that is, compressing a gas makes it hotter. During the compression or pressurization phase of a hyperbaric treatment the temperature inside the chamber can become uncomfortably warm if the airflow is not managed properly.
Dalton’s Law states that in a mixture of gases, the sum of the partial pressures of the gases will equal the total pressure (FIGURE 18-13). Air comprises 21% oxygen and 79% nitrogen. It is well accepted that atmospheric pressure equals 760 mmHg. Using Dalton’s Law, oxygen at 21% has a partial pressure of 160 mmHg and nitrogen at 79% has a partial pressure of 600 mmHg, yielding a total system pressure of 760 mmHg. All gas mixtures obey this same principle.
Henry’s Law states that the partial pressure of a gas dissolved in a liquid is equal to the partial pressure of that gas exerted on the surface of the liquid at equilibrium (FIGURE 18-14). Gases can be dissolved in liquids. If a constant pressure is applied to a liquid and gas solution, the gas will remain in solution. Higher pressures allow increased volumes of gas to be dissolved into the same volume of liquid. If the pressure on the system or solution is lowered, the gas will begin to come out of solution, and form bubbles. This law describes the oxygen-carrying ability of plasma at increased atmospheric pressures. The plasma portion of blood, under sea-level conditions, carries only 0.3 mL of oxygen per 100 mL of blood, in contrast with hemoglobin, which carries 20 mL of oxygen per 100 mL of blood.
In the past, measurement of pressures in the hyperbaric environment has been disputed. In America, it is widely accepted that atmospheres absolute (ATA) is the unit of measure to describe ambient pressure in the hyperbaric environment. FIGURE 18-15 illustrates the relationship of ATA and other commonly used units of measure: millimeters mercury (mmHg), pounds per square inch (PSI), and feet of sea water (FSW). The maximum pressure typically utilized in clinical HBO2 is 3 ATA.
The relationship between the ambient pressure in the hyperbaric chamber and the partial pressures of inspired oxygen and air when patients breathe these two gases is also an important concept (FIGURE 18-16). According to Dalton’s Law, the partial pressure of oxygen at sea level is illustrated by the simple equation (.21 × 760 mmHg) = 160 mmHg, where .21 is the percentage of oxygen in air at sea level and 760 mmHg is atmospheric pressure. As the pressure increases in the hyperbaric chamber, the effective concentration of inspired oxygen increases as a function of pressure even though the partial pressure of oxygen in air remains constant at 21%. The numbers in the bottom part of the figure show the inspired gas pressure when the patient breathes 100% oxygen in the chamber. The magnitude of inspired oxygen concentrations possible within a hyperbaric environment is one reason that accepted guidelines in the use of HBO2 therapy must be followed.
The physiologic effects, or mechanisms of action in hyperbaric oxygenation, are divided into two categories: the mechanical effects of reducing bubble size (occurring in decompression sickness or iatrogenic air emboli) and the effects of an increased partial pressure of oxygen, which vary by organ system and disorder being treated. At higher than atmospheric pressure, oxygen develops pharmacologic properties not present at atmospheric pressure, and thus, like any other drug, oxygen at pressure must be dosed appropriately to avoid complications.
Gas bubbles within the intravascular or tissue compartments, as well as within any closed gas-containing body cavities, are affected by changes in pressure. The effects on bubble or body cavity size are governed by Boyle’s Law, which states that for a given body of gas at constant temperature, the volume of gas is inversely proportional to the pressure. This law and its effect are used to treat any bubble-related disorders.
In the diving and undersea world, decompression sickness develops most frequently when a diver ascends from depth too rapidly, causing dissolved nitrogen to move from the tissue compartment to the intravascular compartment where it forms bubbles. Depending on where the bubbles lodge, they can disrupt blood flow and cause “the bends.” When this process occurs within the musculoskeletal system, it is referred to as type I decompression sickness (DCS I). If this phenomenon occurs within the brain or spinal cord, it is referred to as type II decompression sickness (DCS II), and devastating consequences, such as paralysis and death, may ensue. When an injured diver is recompressed at the surface in a recompression (hyperbaric) chamber, Boyle’s Law predicts that with recompression to 2 ATA, the size of the offending bubbles will be reduced to roughly one-half of their original size (FIGURE 18-17). Typically, with recompression and exposure to higher inspired levels of oxygen, the diver’s symptoms are ameliorated and then, with slow decompression back to sea level, the nitrogen is allowed to remain harmlessly in solution. The bends is a complex disorder, but the principle of recreating the higher pressures found at depth for a diver in a chamber and then very gradually lowering that pressure to sea-level pressure will allow the diver to decompress slowly and the nitrogen to dissipate in a physiologically appropriate and harmless manner.
Another clinical application of hyperbaric therapy is in the urgent treatment of iatrogenic gas embolism. Occasionally, during cardiothoracic or vascular procedures, gas bubbles can inadvertently be introduced into either the venous or arterial system. Depending on the location of the event, the consequences can be mild to severe, as air bubbles within the arterial system can act as thrombotic emboli and lead to stroke-like events.
While in a hyperbaric chamber, dissolved oxygen levels in plasma are dramatically increased more than tenfold. This is enough dissolved oxygen to meet the body’s tissue-oxygen needs and to keep the hemoglobin fully saturated on the venous side of the circulation. This phenomenon was beautifully demonstrated by Dutch surgeon Ite Boerema in a 1960 study entitled, “Life Without Blood.” In this study, Boerema exsanguinated pigs inside a hyperbaric surgical suite and replaced their blood with saline. The pigs remained alive and functioning in this environment essentially without any intravascular blood. At the conclusion of the experiment the saline was replaced by the blood and the pigs were returned to their usual normobaric existence.12 This principle has occasionally been used as a life-saving measure for human cases in which blood transfusions are not an option due to religious beliefs.
When under pressure, oxygen acts like a drug—it has pharmacologic properties and physiologic effects dependent on the exposure pressures and the duration of treatment, much like the serum concentrations of various medications that are dependent on drug dose and dosing interval. This principle is of paramount importance when administering HBO2 in order to avoid adverse effects, which can range from very mild to catastrophic. The clinical administration of hyperbaric oxygen is limited to a maximal pressure of 3 ATA when breathing oxygen. Exceeding 3 ATA provides no additional benefit and only increases the toxic effects of oxygen.
Hyperbaric oxygen therapy is physiologically a vasoconstrictor. Bird and Telfer, as long ago as 1965, demonstrated a 20% reduction in flow to the extremities during hyperbaric oxygen breathing.13 They concluded, however, that this was compensated for by the increased oxygen dissolved in the plasma and tissues, thus the tissues remained hyperoxic in spite of reduced blood flow. FIGURE 18-18 illustrates the hyperoxygenation of plasma in the setting of an occluded artery secondary to thrombosis. Dooley and Mehm also demonstrated delivery despite vasoconstrictive reductions in peripheral blood flow as measured in the calf.14 Using laser Doppler flowmetry, Hammarlund et al. demonstrated an oxygen-dose-dependent vasoconstriction in the skin of healthy volunteers while that same response was not seen in the skin surrounding a chronic venous leg ulcer even though the same subject demonstrated the expected vasoconstriction in the fingertip. After healing the venous leg ulcer, the expected dermal vasoconstriction in the area surrounding the wound was seen once again.15
FIGURE 18-18
Oxygen delivery in the presence of an arterial occlusion
When the artery is occluded and red blood cells cannot carry oxygen to the distal tissue, hyperoxygenation of the plasma can provide compensatory oxygen to the tissue distal to the occlusion. This is the basic principle of healing diabetic foot ulcers with HBO2.

The ability of wounds to heal depends on an adequate supply of oxygen to the wound bed. The normal vascular supply to regional tissues and the wounds therein can be impaired in a macrovascular context, such as with peripheral arterial disease, or microvascular as with diabetes. Whatever the mechanism, wounds will not heal without oxygen. A critical step in wound healing is the production of collagen by fibroblasts. The process of forming new microcirculation (angiogenesis), collagen deposition, and the ultimate closure and strength of wounds is dependent upon adequate oxygenation. Angiogenesis is accelerated in ischemic wounds by hyperoxygenation, and healing time is shortened.16
Gas gangrene is a rapidly progressive and life-threatening bacterial disorder caused by the anaerobic, spore-forming, gram-positive rod, C. perfringens. Gas gangrene is usually initiated by the introduction of C. perfringens through a wound. The resultant spread through tissue planes is rapid, leaving necrotic, devitalized tissue in its wake. Hyperbaric oxygen inhibits the α-toxin produced by this bacterium and has a direct bacterial killing effect due to polymorphonuclear leukocyte (PMN) activity.
The survival of skin and tissue flaps has been studied extensively by numerous investigators. This is an area of very real clinical relevance due to the prevalence of surgical flap procedures for coverage of tissue defects. It seems that hyperbaric oxygen therapy increases the viability and ultimate survival of these flaps.17,18 One of the primary mechanisms appears to be the increased production of superoxide dismutase (SOD) in the hyperbaric environment. SOD is an antioxidative defense mechanism and blocks the production of superoxide radicals from xanthine oxidase, which has been measured in increased quantities in preserved tissue flaps. Zamboni et al. demonstrated that hyperbaric oxygenation improved distal microvascular perfusion as measured by laser Doppler in ischemic skin flaps in the rat.19
According to the UHMS, and as stated earlier, there are presently 14 approved indications for the clinical application of HBO2.10 In nearly all applications, HBO2 is an adjunctive rather than stand-alone therapy. FIGURE 18-19 illustrates categorically the applications of HBO2. This section will focus on the physiologic mechanisms and applications for hyperbaric oxygen therapy in wound healing.
It is well accepted and supported by research that wounds need an adequate supply of oxygen in order to heal. When tissue oxygen levels fall below the minimum levels necessary for healing, a number of detrimental effects occur. These include impairments in collagen production, fibroblast migration and proliferation, and reduced response to host infection. Ultimately, the result of these effects is a halt in angiogenesis and cessation of the wound-healing cascade.16
Under normal sea-level conditions, the arterial partial pressure of oxygen in a person breathing air will be about 100 mmHg. Under these conditions, hemoglobin (hgb) is approximately 97% saturated with oxygen. Breathing increases concentrations of oxygen at sea level and, therefore, has little effect on hemoglobin as it is already completely saturated. In a healthy person with a normal level of hemoglobin, the oxygen-carrying capacity is approximately 20 mL of oxygen for every 100 mL of blood, or 20 volumes percent (vol%). In contrast, the plasma portion of blood carries only about 0.3 mL of oxygen for every 100 cc of blood (0.3 vol%), so plasma, at sea-level conditions, makes no significant contribution to the oxygen-carrying capacity of blood. Therefore, under conditions of complete saturation of hemoglobin, the only way to increase the oxygen-carrying capacity of blood is to increase the amount of oxygen dissolved in plasma.
Looking again at Henry’s Law, the partial pressure of a gas dissolved in a liquid is equal to the partial pressure of that gas exerted on the surface of that liquid. Accordingly, as atmospheric pressure increases, so does the concentration of dissolved oxygen in the plasma. When breathing oxygen at 2 ATA, or twice the atmospheric pressure, the arterial partial pressure (pO2) rises to approximately 1400 mmHg, representing over a tenfold increase in arterial oxygen content as compared to that seen at sea level, or 1 ATA. Along with the rise in arterial oxygen content comes a concomitant rise in tissue pO2 levels, which rise to approximately 300 mmHg at 2 ATA. In the final analysis, at 2 ATA, the oxygen-carrying capacity of blood is increased from 20 vol% to about 24 vol%, an increase almost entirely due to an increase in dissolved plasma oxygen (FIGURE 18-20).
The process of wound healing is an elegantly regulated cascade of cellular and biochemical events that work in concert to restore wounded tissue to a prewound condition through the encouragement of physical and physiologic processes. Once wounded, tissue and skin can never be restored to its precise normal anatomic and physiologic state, and tissue and tensile strength will never be as high as in the prewound state, so the ultimate goal of the healing process is to restore tissue to an intact and functional status.
Many studies have demonstrated that wound healing is dependent on adequate oxygenation of the vascularized tissue in the periwound area, the area immediately surrounding a wound. HBO2 intermittently increases the tissue pO2 as well as the diffusing distance of oxygen in this periwound area.20 This increase in tissue pO2 augments wound healing by accelerating fibroblast replication and the resultant collagen synthesis, collagen being the structural building block of new tissue and skin. This in turn leads to the process of granulation, neovascularization, and epithelialization.21
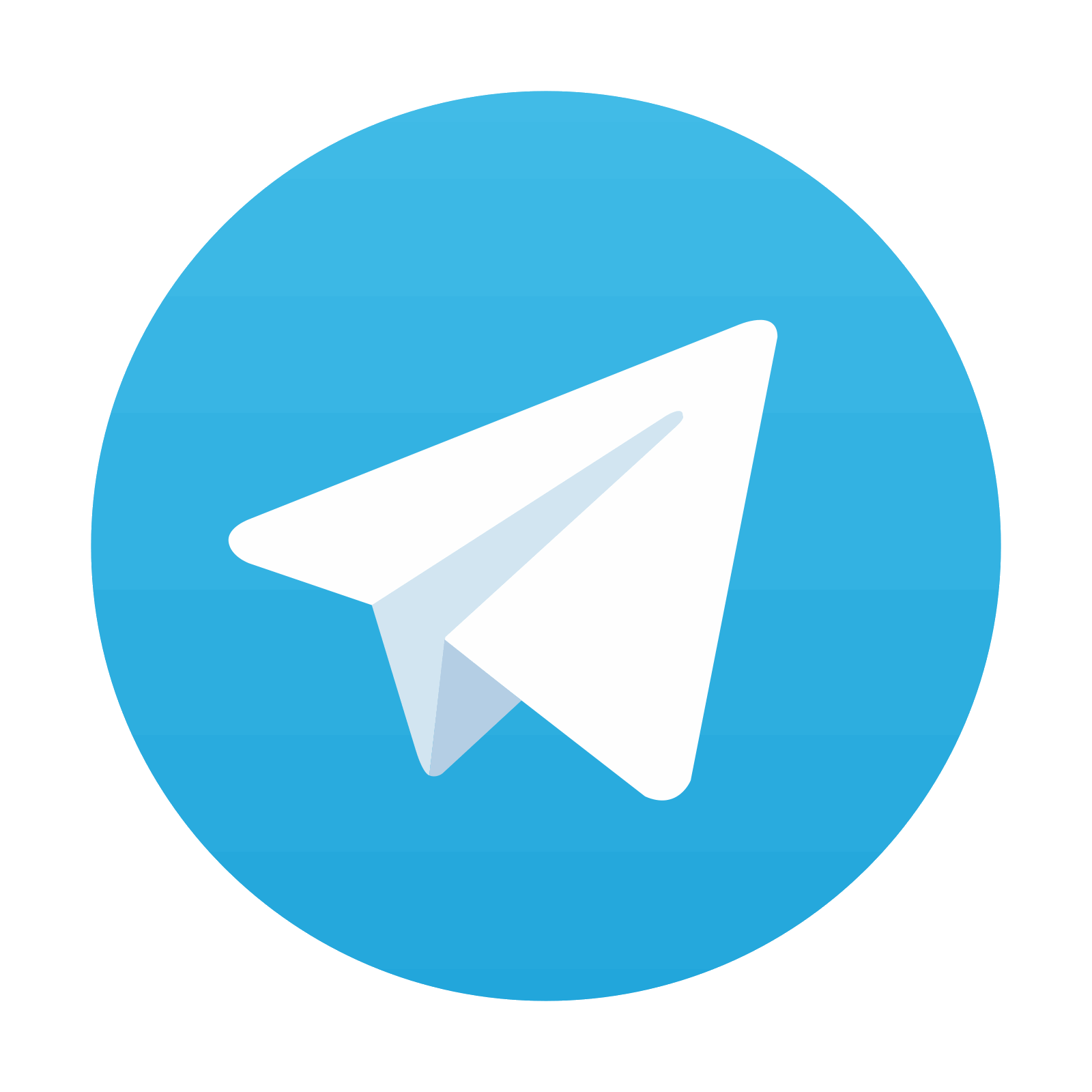
Stay updated, free articles. Join our Telegram channel
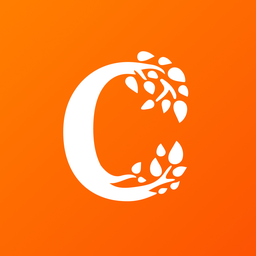
Full access? Get Clinical Tree
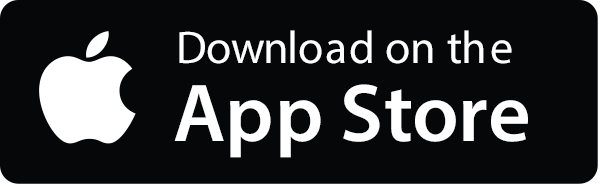
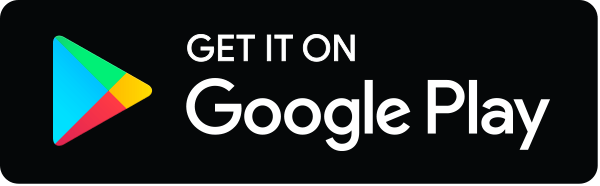
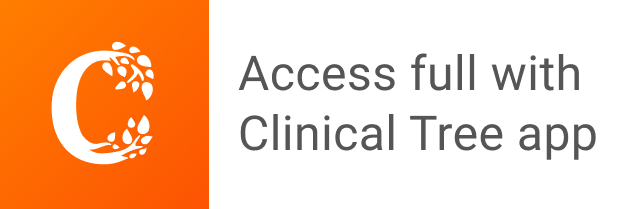