“The most common complication of hand fractures is not malunion or infection. Rather, it is joint contractures and tendon adhesions.”
Baratz and Divelbiss, 1997
- ▪
Fracture reduction
- ▪
Fracture stability
- ▪
Soft tissue mobility
- ▪
Zone of injury (ZOI)
- ▪
Minimally invasive surgery (MIS)
- ▪
Clinical physiological correlation of tissue healing
- ▪
Clinical progression based on fracture healing and soft tissue response
Hand fractures do not occur in isolation. Every hand fracture has a proportionate closed or open injury to and response within its adjacent embedding soft tissues, creating a “zone of injury” (ZOI) , ( Fig. 29-1A ). Immediately after fracture, a central hematoma occurs within the space between the fragments and expands about the fracture. Interstitial edema accumulates within the impacted soft tissues and deposits fibrin as part of the acute inflammatory response to injury. The peripheral margins of the edematous response define the ZOI ( Fig. 29-1B–D ).

Initial local signs of hand fractures include primary bone deformity, secondary joint deformities, motion and instability at the fracture site, pain, tenderness, swelling, ecchymosis, and heat. Without treatment, all of the tissues encompassed within the ZOI tend to become progressively bound into a single amalgam (one wound/one scar) by the fibrin, fibronectin, and mucopolysaccharides (“tissue glue”) contained in the extracellular matrix (ECM) , ( Fig. 29-2 ). Joints adjacent to the fracture are often affected and can develop permanent intrinsic or extrinsic (or both) adhesions, stiffness, and deformity. Pseudoclaw deformities may develop ( Fig. 29-3 ). Tendons tend to adhere to adjacent structures, sometimes impairing gliding. Progressive stiffness, atrophy, dysfunction, and pain may result (“fracture disease”).


The essence of hand fracture management is restoration of anatomic osseous integrity and stability throughout fracture healing; together with simultaneous preservation of the normal length and elasticity of the ligaments and joint capsules; and facilitation of early independent joint motion, tendon gliding, and functional recovery of the hand and digits. Gentle, progressive, controlled, passive range-of-motion (PROM) and unresisted active range-of-motion (AROM) exercises should be initiated as soon as the fracture is reduced and stable. Early motion stimulates parallel interstitial collagen fiber alignment, which enhances scar elasticity and prevents or disrupts early adhesions in the interstitial spaces between disparate tissues during concurrent fracture healing and joint and tendon rehabilitation. The more severe the injury, the more extensive and proliferative the fibroblastic response; and thus, the more compelling early motion becomes. The duration and intensity of exercises are gradually advanced in accordance with the stability of the fracture, the response of the soft tissues, and the patient’s pain tolerance. The resting hand and digits should be positioned and protected in the functional (intrinsic plus) “safe” posture between exercise sessions to prevent joint contractures and central slip attenuation.
Fracture and soft tissue healing are processes, not events that occur over time. Bone healing and scar formation undergo three discrete yet artificial and overlapping stages of progression: the acute or inflammatory phase, the reparative or proliferative phase, and the remodeling or maturation phase. Knowledge of the molecular and cellular interactions throughout the stages of bone healing and scar formation, the timing of events, and how these processes simultaneously interrelate within and adjacent to the common environment of the ZOI allows the physician and therapist to correlate incremental clinical functional rehabilitation with cellular and molecular events and tissue recovery. The physician, therapist, and patient must work together as a committed team to achieve the best outcome possible.
Fracture Disease
Immobility leads to so-called fracture disease. Fracture disease begins with uncorrected deformities of bone and joints, acute pain, and persistent edema. Without intervention, fracture disease evolves to include stiffness, tendon adhesion, capsular and ligamentous contracture or attenuation, central slip attenuation, infiltrative scar formation, and pseudoclawing. Additional edema may occur due to limb dependency, tight dressings, and pressure points over bone or joint prominences. Consequently, fracture disease may spread beyond the ZOI and become complicated by local or regional chronic complex regional pain (CCRP, reflex sympathetic dystrophy [RSD]). Joint deformities may become progressively fixed as early as 3 to 6 weeks after injury, and CCRP (RSD) may develop. Pain may become chronic, disproportionate to expectations, and unresponsive to ordinary treatment. Algodynia and hypersensitivity may occur. If undeterred, trophic changes gradually develop, including extensive fibrosis, brawny skin induration, shiny skin, digital tapering, loss of rugal pattern, hair loss, nail growth changes, temperature and sweating abnormalities, and local or regional osteopenia. Fracture disease and CCRP become progressively recalcitrant to treatment over time. Therefore, early intervention with fracture stabilization and digital exercises is essential.
Fracture Management
Ensuring anatomic fracture position and stability are the primary initial goals of hand fracture management. Hand fractures that are undisplaced and inherently stable do not require reduction, but do need some protection against deforming forces and additional impact during early healing. A small cohort of hand fractures have inherent stability following manipulative reduction. They also require the use of a protective static or functional orthosis for 3 to 4 weeks. The remaining hand fractures require closed or open reduction and either temporary or permanent implant fixation to restore their anatomic integrity and stability as fracture healing and rehabilitation progress.
Early fracture reduction and stability control pain, restore fracture surface apposition and the normal proportionate relationships between bone and adjacent soft tissues, promote bone healing, and provide a platform both for any necessary soft tissue repairs and for timely rehabilitation. Function follows form. Stability prevents “false motion” at the fracture site and allows revascularization and healing of repaired tissues to proceed without disruption. Fracture fixation should be as “atraumatic” as possible to prevent or limit additional bone devascularization, soft tissue damage, and fibroplasia. Subperiosteal dissection, flexor tendon sheath injury, and extensor tendon injury over the proximal finger phalanges in particular, stimulate additional fibroplasia.
Initial Treatment
Definitive fracture treatment may be delayed for up to 5 to 7 days in closed, low-energy hand fractures having two fragments with little alteration of outcome. Open fractures require urgent attention to débride, cleanse, and catalogue the wound; reduce the fracture; provide at least provisional fracture stabilization; and prevent infection. Simple (two-part) fractures with clean, limited skin lacerations may be safely and definitively stabilized and the skin repaired primarily. Soiled and complex wounds should initially be left open and then ideally reassessed within 48 hours. Additional cleansing and debridement performed at that time should render most wounds clean and suitable for definitive bone, tendon, and nerve and skin repair. , If not, the cleansing, debridement, and wound assessment may be repeated at 24- to 48-hour intervals until the wound is rendered clean. The least invasive method of internal fixation should usually be selected from equally applicable methods.
Reduction
Although limb length, appearance, and function were probably somewhat impaired, primitive humans and animals in the wild have successfully healed markedly displaced, untreated fractures ( Fig. 29-4 , online). Early indirect fracture healing evolved in an environment where mobility favored survival. The need to secure nourishment and avoid predators resulted in motion about the fracture site that often stimulated callus formation. Over time it became evident that fractured bones held in anatomic reduction healed with better outcomes. The oldest record of fracture reduction is found in Egyptian hieroglyphics (2450 bce ) depicting orthoses made from papyrus that were used to reduce and support distal forearm fractures ( Fig. 29-5 , online).


Shortening, angulation, and rotation are the principal elements of deformity and may be seen individually or in combination. Alterations of bone anatomy may affect functional outcome commensurate with their severity. Reduction restores the fracture fragments to their prefracture anatomy. Although a perfect reduction is of course ideal, the hand and digits have a remarkable capacity for functional adaptation to and tolerance of small and, sometimes, greater fracture deformity.
Anatomic Parameters and Functional Correlations
Recent reports have illuminated the correlation between deformity and functional loss and may help the physician to decide whether to accept or correct a residual deformity. Hand dominance, occupation, or special individual needs may play a role. Observation of and discussion with the patient and the timing of presentation may also be critical in the decision-making process. The risk of postoperative digital stiffness may outweigh the benefit of lesser gains in anatomic fracture restoration in instances of small deformities, particularly in late-presenting inveterate fractures that have the early formation of a soft or hard callus that prevents closed manipulative fracture reduction.
Metacarpal Fractures
Metacarpal (MC) shaft fractures tend to angulate dorsally, owing to the unbalanced pull of the interosseous muscles and extrinsic finger flexors on the distal fragment ( Fig. 29-6 ). Intrinsic muscle shortening and altered muscle tension dynamics lead to increasing grip weakness after 30 degrees of dorsal MC angulation. There may also be loss of knuckle contour, pseudoclaw deformity, and a palpable or even visible MC angular deformity on the dorsum of the hand, and prominence of the MC head in the palm of the hand. The ring and small finger MCs are more tolerant of dorsal angulation than those of the index and middle fingers due to their increased carpometacarpal flexibility. Satisfactory results have been reported with as much as 70 degrees of dorsal angulation of subcapital MC (boxer’s) fractures. ,

Approximately 7 degrees of extensor lag may develop for each 2 mm of residual finger MC shortening after fracture healing. The intermetacarpal ligaments usually prevent greater than 3 to 4 mm of shortening of finger MC fractures. Internal MCs (third and fourth) have more restraint than border MCs (second and fifth) since the distal fragment is anchored by intermetacarpal ligaments on both sides of the MC head. The intrinsic hand muscles contribute 40% to 90% of grip strength. , As great as 8% loss of grip power may result from every 2 mm of MC shortening. Based on this information, finger MCs may tolerate as much as 3 to 4 mm of shortening, and occasionally more, with only minimally noticeable clinical deformity and functional loss. In individual instances, surgeons may choose to accept 3 to 4 mm or perhaps more shortening as an alternative to the risks of scarring and stiffness from surgical intervention.
Lateral MC angulation of 5 to 10 degrees may be tolerated, provided that no significant finger impingement occurs during motion. MC angulation may be best observed with the fingers straight, whereas impingement becomes more apparent as the fingers progressively flex.
The intermetacarpal ligaments between the MC heads provide some rotational stability to the distal fracture fragment. The internal MCs have more restraint than border MCs. Rotational deformity greater than 5 degrees may result in finger impingement, or “scissoring” (overlap). , Seitz and Froimson reported that 10 degrees of MC malrotation resulted in 2 cm of overlap at the fingertips. Rotational deformity of the fingers may be apparent with full digital extension, but becomes progressively more pronounced as the collateral ligaments tighten with finger flexion.
Phalangeal Fractures
Displaced fractures of the proximal phalangeal shaft characteristically display an apex palmar angulation (see Fig. 29-6 ). The intrinsic muscles flex the proximal fragment, whereas the attachment of the central slip to the dorsal proximal border of the middle phalanx extends the distal fragment. The axis of rotation of proximal phalangeal fractures lies on the fibro-osseous border of the flexor tendon sheath. The distance of the moment arm from the rotational axis of the fracture site to the extensor tendons is greater than that between the axis and the flexor tendons, further contributing to apex palmar angulation. Coonrad and Pohlman reported limitation of finger flexion with palmar angulation of greater than 25 degrees in extra-articular phalangeal fractures at the base of the fingers and advocated correction of greater deformities. More recently, Agee reported shortening of the dorsal gliding surface of the proximal phalanx relative to the length of the extensor mechanism if volar proximal phalangeal angulation exceeds 8 to 15 degrees. As palmar angulation incrementally shortens the fractured proximal phalanx, the extensor mechanism may have as much as 2 to 6 mm of reserve, owing to its viscoelastic adaptive properties, before the sagittal bands tighten to produce a progressive extensor lag at the proximal interphalangeal (PIP) joint of an average of 12 degrees for every millimeter of bone–tendon discrepancy. Skeletal alignment sufficient to prevent or minimize extensor incompetence at the PIP joint and to restore the floor of the flexor tendon sheath is critical in the effort to restore both extensor and flexor tendon gliding and function. A persistent palmar angular deformity of the proximal phalanx may lead to permanent attenuation of the central slip, extensor lag, and pseudoclawing of the finger that may persist despite later correction of the osseous deformity. Apex palmar deformity of middle phalangeal fractures manifests similar problems with skeletal shortening, extensor lag of the distal interphalangeal joint, and alteration of flexor tendon gliding and dynamics.
Stability
Although functional recovery (motion) is the ultimate overall goal of hand fracture management, stability is the ultimate purpose of reducing fracture fragments. Fractures that do not displace spontaneously or with unresisted exercises are considered stable. Inherently stable fractures safely tolerate incremental tendon gliding and joint motion, maximizing their functional recovery. Reduced fractures that displace spontaneously or with unresisted motion cannot maintain their alignment. These fractures require some form of additional support or fixation to ensure that correct anatomy is maintained during fracture union. Fracture fixation methods vary from external to internal support. Miniscrews, miniplates, tension band wiring systems, and “90-90” wiring provide secure fixation and allow healing to occur by direct bone formation. Less secure, “flexible” fixation methods, such as Kirschner wires (K-wires) and external minifixators, internally fix fractures. “Flexible” fixation allows nondisruptive micromotion at the fracture site, thus supporting and stimulating indirect bone healing. Flexible fixation permits sufficient exercising to prevent tendon adhesions and joint contracture in most instances. Exercises must initially be restricted in their arc and intensity to prevent loss of reduction, wire migration, or skin and soft tissue irritation. Balancing the need to maintain fracture stability with the safe introduction of motion is based on scientific predictability and yet is the art and craft of hand fracture management.
Fracture configuration, impaction, periosteal disruption, muscle forces, and external forces may influence stability. Transverse and short oblique fractures have a stable conformation, whereas long oblique and comminuted fractures and those with bone loss have unstable patterns. Periosteal disruption correlates with the severity of the injury and the amount of fracture displacement. An intact or restored periosteum or bone impaction can support fracture alignment. Unbalanced muscle or external forces can cause fracture displacement or collapse. The “safe” hand position neutralizes the effects of muscle forces at the fracture site and thus, enhances fracture stability.
Fracture Stabilization
Nonoperative Management
Stable Undisplaced or Minimally Displaced Fractures
A majority of closed extra-articular hand fractures are simple (two-fragment), undisplaced or minimally displaced, and stable, and therefore may be safely and effectively treated by minimal protective orthoses and early motion. This is especially true of impacted transverse or short oblique fractures at the base of the proximal phalanx and subcapital MC fractures with no rotational malalignment. , Indirect (secondary, enchondral, “biological,” “physiologic,” or undisturbed) bone healing occurs.
Displaced Fractures That Are Stable After Closed Reduction
Closed displaced transverse or short oblique simple extra-articular hand fractures may often be successfully reduced by closed manipulation (closed reduction, CR) and heal indirectly, or biologically. Fractures with simple angulation are typically more easily reduced. The cortices on the angulated side of the fracture act as a hinge to implement reduction. The periosteum may be sufficiently intact to contribute to stability following reduction. Distal proximal phalanx fractures may have tenuous stability owing to their diminished cross-sectional area and the leverage of muscle forces at the fracture site. Successfully reduced fractures may require static immobilization for 3 weeks. External orthoses that protect the fracture and allow early ROM are optimal ( Fig. 29-7 ).

Internal Fixation
Indications
The physician must weigh the risks to benefits of nonoperative versus operative correction and stabilization for each fracture and deformity. Internal fixation is reserved for unstable fractures, irreducible fractures, lost closed reductions, comminuted fractures, fractures with bone loss, multiple hand fractures, hand fractures accompanied by ipsilateral extremity injuries, open fractures, and pathologic fractures. Secure stabilization may be advantageous for polytraumatized patients with a lower extremity long bone fracture to facilitate patient treatment, transfers, and the use of crutches or a walker for ambulation. Hall has reported the advantages of using secure fixation in noncompliant patients. Maintenance of anatomic fracture position, wound access, early edema management, and the ability to initiate early and intensive exercises are relative benefits of secure internal fixation.
When surgery is necessary, the physician must also choose among approaches and implants. Fracture implants do not necessarily have to be the strongest available, but rather should be adequate in strength to reliably hold the fracture through the early stages of fracture callus formation and maturation.
Minimally Invasive Surgery
Hand surgeons have long known the perils of indiscriminate open reduction and internal fixation and have been among the first to encourage minimally invasive surgery (MIS). MIS with closed reduction and internal fixation (CRIF) preserves periosteal integrity and circulation at the fracture site, minimizes expansion of the ZOI, allows for biological (undisturbed) fracture healing, and reduces the risk of additional adherent scar formation. , CRIF is advocated for unstable simple closed hand fractures whenever possible. CRIF aligns the fracture fragments; avoids periosteal dissection, bone exposure, and disturbance of the blood supply; minimizes implant-to-bone contact; allows physiologic interfragmentary stress and strain; stimulates callus formation; and allows graduated exercise.
Transverse or short oblique phalangeal and MC fractures may be stabilized with single or multiple intramedullary wires. Locking pins or subcortical buttressing of the wires at one or both ends of the fragments adds stability at the fracture site. Oblique closed diaphyseal phalangeal fractures may be stabilized with percutaneous transfixation wires. , Percutaneous wires may be inserted transversely through an intact adjacent MC into one or both fragments of a closed simple unstable MC fracture to provide fixation. Complex pilon fractures at the PIP joint can be reduced and the joint structure remodeled with the MIS percutaneous application of a transverse wire through the middle phalanx. The wire is dynamically attached to a moving component on an orthosis. Traction reduces the fracture length, while the intact and stretched periosteal sleeve compresses the fracture fragments ( Fig. 29-8 ).

Open Reduction and Internal Fixation
Stable, or rigid, fixation is achieved at the price of a second planned wound to address the initial fracture injury. Sharp, low-energy, neutrally placed incisions are less traumatic than random, high-energy, blunt impact, but they nevertheless create additional fibroplasia. The greater potential for soft tissue scarring with open reduction methods may be partially offset by the opportunity for earlier and more intensive exercise.
Most hand fractures are approached with a dorsal incision. Surgeons should try to avoid violation of the peritenon between the skin and tendon and between the tendon and bone as much as possible. Incising the skin and MC periosteum to one side of the extensor tendon and placing retractors between the periosteum and the bone helps to accomplish this goal. Neutrally placed midlateral incisions and lateral band retraction or excision may facilitate phalangeal exposure for selected proximal phalangeal fractures, minimizing the risks of tendon attrition, scarring, and intrinsic tightness that may occur with lateral band incision and repair over an implant. Open fractures may often be approached by extending the laceration or wound. It may be prudent to secure the fracture with the most stable available implants that resources and the fracture configuration and operative exposure will allow.
Two or more miniscrews may be used to secure and often compress an oblique diaphyseal fracture. Miniscrews may be thought of as small straight K-wires with a head and threads. The screw head buttresses the adjacent cortex while the threads grip the opposite cortex and enhance stability by compressing the fracture. The miniscrews protect each other from shear, rotational, and bending forces during rehabilitation and have the additional advantage of remaining in place for the duration of fracture healing. Miniscrew fixation supports primary bone healing. Miniscrews are usually removed only if they become symptomatic.
Although the dissection required for plate application, particularly in closed fractures, is technically demanding and may devascularize adjacent bone, delay bone healing, and generate additional fibroplasia, miniplates that compress the fracture provide excellent stability at the fracture site throughout healing. In open fractures, especially those with complex wounds, comminution, or bone loss, priority must often be given to anatomic fracture restoration and healing. Secure miniplate fixation provides for earlier and more intensive exercises than does flexible fixation and may at least partially offset the disadvantages of operatively generated fibroplasia. “To operate on a fracture and fail to take advantage of the opportunity for early motion exposes the patient to the worst of both worlds: the injury is compounded and the potential gain is squandered.”
Miniplates positioned dorsally under the extensor tendons or dorsal apparatus may restrict the extremes of digital motion by their physical presence, especially as their edges approach adjacent joints. This is especially true of miniplates applied on the proximal phalanx adjacent to the PIP joint.
Straight miniplates are customarily applied to mid-diaphyseal fractures. Mini condylar plates have a fixed angle blade or locking peg that may assist in fracture reduction and may be applied either dorsally or laterally for juxta-articular fractures. , Primary or delayed primary bone grafting may be performed at the time of plate application in most patients. Puckett and colleagues reported that microplates that allow closure of the periosteum have improved results in phalangeal fracture plating compared with larger miniplates that prevent periosteal closure. Fracture stabilization, bone grafting when necessary, wound closure or coverage, and the initiation of rehabilitation within 3 to 5 days of injury may provide the best opportunity for optimal functional recovery of open or operated hand fractures.
Pathophysiology
Regeneration is the restoration of damaged tissue with cells of the same kind. Although some species such as lizards (e.g., salamanders) and starfish can regenerate entire lost limbs, humans have retained this potential in only select tissues, liver, and bone, and to limited degrees. Peripheral nerves may regenerate distal to the cell bodies, and superficial skin lacerations regenerate true epidermis. Most soft tissue injuries heal instead through a process of repair , in which fibrous scar tissue forms to weld the damaged cells. Scar, then, is the biological “glue” that allows tissues to heal that have lost their regeneration potential. Bone heals by either primary or secondary regeneration. If the fragments are too distant from each other or insufficiently stable, regeneration cannot occur. , Conversely, small degrees of nondisruptive cyclic compression at the fracture site stimulates secondary bone regeneration and, later, bone remodeling.
Bone regeneration and soft tissue repair depend on complex processes—mechanoreception and cell signaling—to recruit migration of progenitor cells to the wound and to modulate proliferation and differentiation of these multipotential mesenchymal cells into cells capable of forming bone or scar tissue. This newly formed tissue must mature, strengthen, and reform its configuration to duplicate or approximate the original architecture and tissue function.
Bone must eventually heal with new bone by either passing through a temporary fibrocartilagenous phase prior to ossification (indirect healing) or through direct osteonal regeneration across the fracture site (direct healing). Both types of fracture healing ultimately lead to the regeneration of strong mature lamellar bone.
Indirect Bone Healing
Indirect fracture healing has also been called secondary healing, healing by enchondral ossification, or biological (undisturbed) bone healing. Indirect bone healing evokes an evolutionary design wherein the requisite cellular response is matched to the wound environment to ensure that the repair process leads to bone formation. A fusiform external callus surrounds the periphery of the fracture site and cascades through the entire spectrum of connective tissue from hematoma to granulation tissue, fibrous tissue, hyaline cartilage, woven bone, and, ultimately, lamellar bone to weld the fracture fragments and ultimately allow cancellous and medullary healing. Five factors are necessary for indirect bone regeneration: (1) a fracture hematoma as a source of signaling molecules, (2) a vibrant, diversified cell population to ensure healing progression and nutrition, (3) an adequate blood supply, (4) an evolving scaffold for cellular differentiation, and (5) a mechanical environment of relative stability that minimizes interfragmentary strain. , This programmed sequence of tissue differentiation allows the most robust cells—fibroblasts and chondroblasts—to endure through an environment of compromised circulation, oxygen depletion, and instability until the metabolic and mechanical conditions are conducive to successful osteoblastic bone replacement. Finally, fragile woven bone is stress-remodeled into strong lamellar bone. Cyclic reciprocal micromotion (compression–distraction) facilitates this process.
Inflammatory (Acute) Phase (Time of Injury to Day 5)
The bones of the hand have a rich periosteal, intramedullary, and metaphyseal blood supply. No cell lies more than 300 µm from a blood vessel. Histamine-mediated vasodilation occurs during the first several minutes following fracture. Whole blood pours into and expands the space about the fracture fragments and torn soft tissues, creating a liquid hematoma ( Fig. 29-9A , online). Local compression, secondary vasoconstriction, platelets, and the clotting cascade lead to the formation of a gelatinous blood clot. There are no functioning blood vessels in the fracture clot.

The hematoma and injured periosteum have important and complementary roles in the cellular events necessary for normal fracture healing. Consequently, early evacuation of the hematoma or excision or stripping of the damaged periosteum retards indirect healing. The blood clot contains growth factors, cytokines, and other complex peptides that stimulate neoangiogenesis and attract white blood cells, macrophages, and multipotential mesenchymal cells to the fracture area. Within 24 hours after fracture, endothelial cells that line intramedullary capillaries enlarge and migrate into the damaged area, initiating neoangiogenesis. Cells, nutrients, and cell-signaling peptides diffuse into the periphery of the blood clot from local soft tissues and new capillaries. White blood cells secrete lysozymes that dissolve the blood clot. Macrophages and osteoclasts phagocytize the clot and necrotic debris. Macrophages and platelets generate many of the additional complex cell-signaling peptides that modulate mesenchymal cell differentiation and tissue development. Mesenchymal cells recruited from the damaged periosteum, adjacent muscle, and regenerating blood supply differentiate into fibroblasts in the initially acidic and hypoxic environment and invade the periphery of the blood clot. The periosteum is the principal source of multipotent mesenchymal cells. Granulation tissue (fibroblasts and white blood cells) form the early pliable fusiform external fracture callus on the periphery of the blood clot and between the fragments.
Reparative (Proliferative) Phase (Day 5 to Day 21)
Healing by natural means (indirect or secondary repair) involves a gradual substitution of tissues that protects the fracture while bone is regenerated. Tissue oxygenation gradually improves and acidity proportionally decreases as neoangiogenesis progresses, facilitating centripetal healing of the external fusiform fibrous fracture callus from its periphery toward the center of the fracture. Mesenchymal cells now differentiate into chondrocytes rather than fibroblasts, until the fibrous callus is replaced by nonmineralized hyaline cartilaginous scaffolding that encompasses the fracture site ( Fig. 29-9B , online). If the bone gap is not bridged by cartilaginous callus within 2 to 3 weeks after injury, the initial callus response may fail.
As stability increases, tissue oxygenation continues to improve and the ECM becomes alkaline. At 10 to 21 days after injury, the cartilage matrix begins to undergo progressive centripetal enchondral ECM calcification. Bone morphogenic protein 7 (BMP-7), also known as osteogenic protein 1 (OP-1), modulates new mesenchymal precursor cells to become osteoblasts. The new osteoblasts invade the cartilaginous callus to form immature trabecular “woven bone” across the fracture site. Cartilage cells undergo apoptosis and are removed by chondroclasts. From the second to the sixth week after injury, intermittent axial compression can increase corticomedullary blood flow and stimulate chondrogenesis and, later, osteogenesis throughout the callus. Conversely, excessive shearing motion during this time can impede neoangiogenesis and delay or prevent fracture healing by damaging new arterioles and capillaries. Throughout this process, the external fracture callus progressively solidifies and becomes stronger. The periosteal, intracortical, cancellous, and intramedullary blood flow normalizes. Intramedullary healing proceeds. Interfragmentary motion (IFM) progressively decreases and finally ceases at approximately 4 to 6 weeks after injury. At this point the fracture is clinically stable, even though radiolucent portions of the fracture may be seen on radiograph.
Remodeling (Maturation) Phase (Day 21 to 18 Months)
The reparative phase of healing extends into the remodeling phase. The initially accreted immature woven bone is randomly aligned and weaker than normal lamellar bone. The ideal goal of fracture healing is to regain the original form and function of the healed bone ( Fig. 29-9C ). Progressive remodeling of primary immature trabecular bone along the lines of axial stress forms compact lamellar bone (Wolff’s law) and reestablishes the Haversian canals. During this process, osteoclasts remove the weaker woven bone from the tension sides of trabeculae, and osteoblasts accrete linear layers of mature new bone on the compression sides parallel to the stress forces. Osteoclastic metabolism (bone resorption) is initially 50 times greater than osteoblastic activity (bone accretion). This initial imbalance creates temporary local bone porosity that facilitates neovascularization into the Haversian canals. Bone remodeling and metabolic equilibration takes approximately 5 months to complete in the tubular bones of the hand. These forces direct the orientation of newly formed bone to provide better mechanical advantage and duplicate the original shape and strength of normal bone. Each region of each bone responds to a particular amount of intermittent strain, creating an “optimal strain environment” for remodeling. , Static loading has little influence on bone architecture. A distinctive “dose/response” curve is generated by daily short, intermittent stimulation exposure with strain magnitudes within the physiologic safe range for the bone. Fracture remodeling is optimally influenced by a regimen of dynamic, diverse activities that do not exceed the strain tolerance level of the bone. Remodeling does not always occur simultaneously in all areas of the fracture. The injured bone regains approximately 80% of its original strength within 3 months after fracture, although 18 to 24 months is often required to regain normal tensile strength.
Remodeling allows some correction of angular deformities along the lines of linear stress in younger children, and occasionally in adolescents and adults, but does not correct rotational deformities. The ability of bone remodeling to correct angular deformities by remodeling diminishes with age.
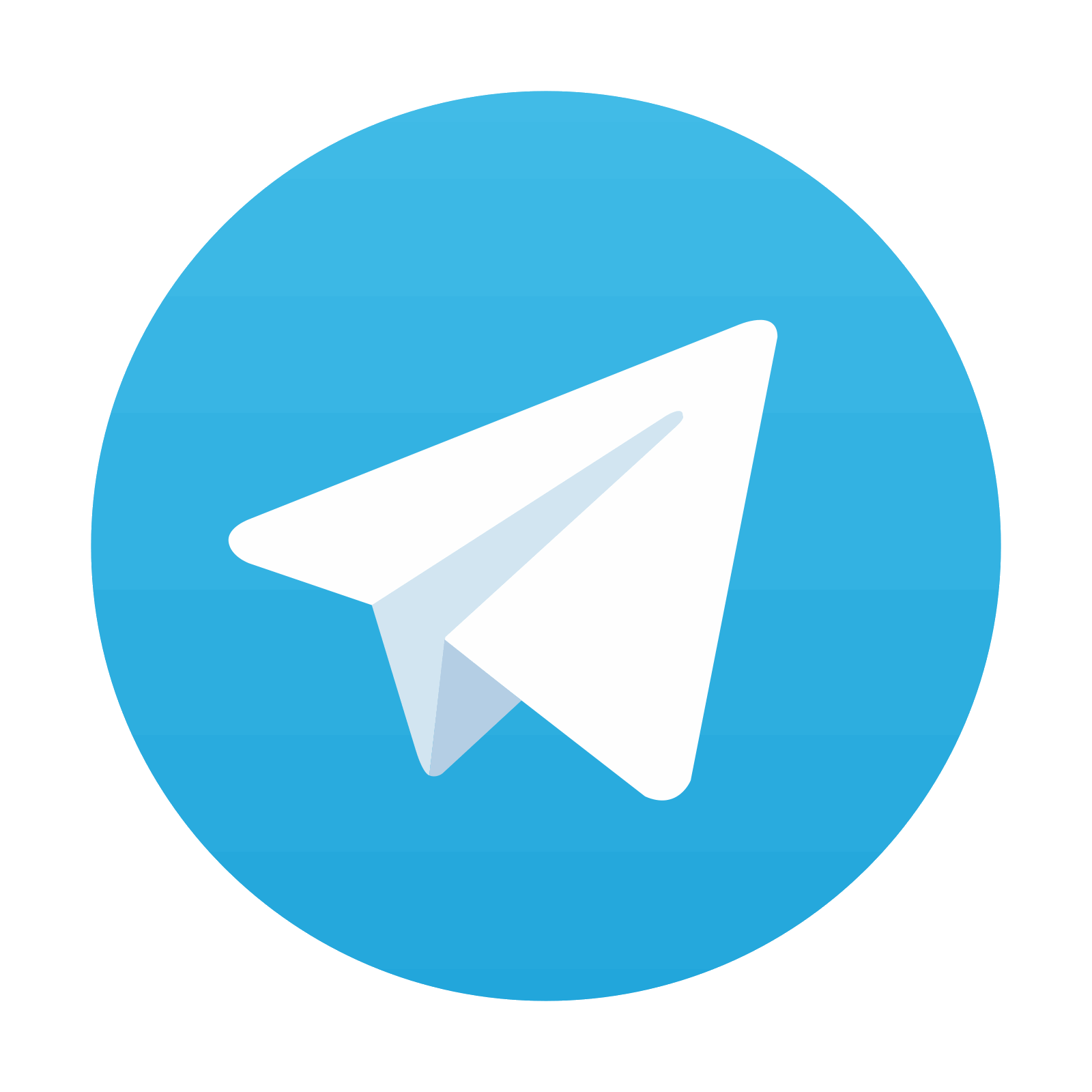
Stay updated, free articles. Join our Telegram channel
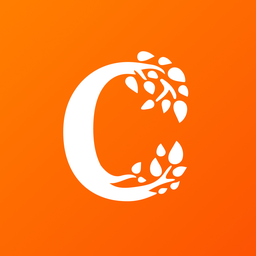
Full access? Get Clinical Tree
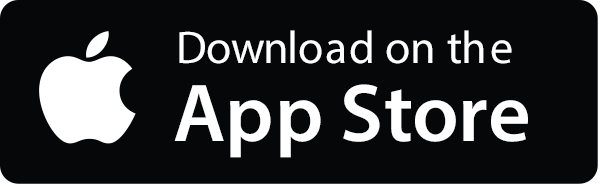
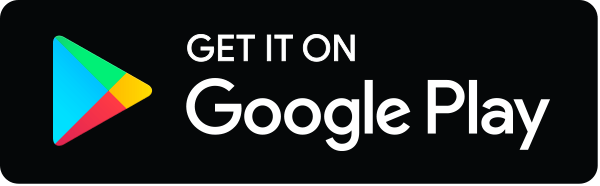