Growth Plate Anatomy
Hannah-Noemi Ladenhauf
Gerd Seitlinger
THE DEVELOPMENT OF BONE
During fetal development, three distinct ways of differentiation of the skeleton have been described. Somites, bilaterally paired blocks of mesoderm that form along the anterior-posterior axis, give rise to the axial skeleton. The lateral mesodermal plate generates the limb skeleton, and the cranial neural crest develops the branchial arch, craniofacial bone, and cartilage.
The formation of new bone tissue by osteoblasts is termed ossification or osteogenesis. In the process of generating new, healthy bone, there are two major pathways: intramembranous ossification and endochondral ossification. Both involve the transformation of a preexisting mesenchymal tissue into bone tissue. Intramembranous ossification occurs when flat bone needs to be built. It is an essential process during the formation of rudimentary bones of the skull.
The first step during intramembranous ossification is the formation of bone spicules, which will subsequently fuse with each other. Therefore, a small group of mesenchymal precursor cells proliferate and form a dense cluster of cells, a nodule. The cells within the nodule replicate and develop into an osteoprogenitor cell.1 During further development, the osteo-progenitor cell changes in morphology and displays the morphologic characteristics of an osteoblast. Osteoblasts can then create an extracellular matrix containing type 1 collagen fibrils, termed osteoid, an immature bone, which increases the size of the bone spicules. As the spicules continue to grow, they fuse and form trabeculae. Some of the osteoblasts become incorporated within the osteoid and develop into osteocytes, which can later mineralize the immature bone tissue. As growth continues, the trabeculae become interconnected and so-called woven bone is formed. This initial trabecular network is also termed primary spongiosa. The periosteum is formed around the trabeculae by differentiating mesenchymal cells. Osteogenic cells that originate from the periosteum increase appositional growth and a bone collar is formed. The bone collar is then mineralized and lamellar bone is formed. Unlike endochondral ossification, intramembranous ossification does not require the preexistence of cartilage.
Endochondral ossification is the second process by which new bone tissue can be created. This pathway requires the formation of a cartilaginous template, which can then be converted into bone. This process occurs during embryonic long bone formation and longitudinal physeal growth. Endochondral ossification does not only occur at the growth plates but also at many other sites. Epiphyses and their equivalents including carpals, tarsals, sesamoids, and apophyses all develop solely by endochondral growth.2
The pathway of endochondral growth can be divided into several stages. First, mesenchymal cells differentiate into chondrocytes, which grow cartilage. The chondrocytes will then proliferate rapidly to form the model for the bone. The cartilage will grow in length by successive cell division of chondrocytes, accompanied by continuous secretion of extracellular matrix. This process is termed interstitial growth. In contrast, appositional growth happens when the cartilage model also grows in thickness through addition of more extracellular matrix on the peripheral cartilage. In a later stage, the chondrocytes will terminate their division process and increase dramatically in volume, becoming hypertrophic. This alters the matrix and thus enables it to become mineralized by calcium carbonate and to be invaded by blood vessels. The hypertrophic chondrocytes will later go into apoptosis,3 leaving this space to become bone marrow. So-called stacked cells which earlier surrounded the cartilage model now give rise to the lineage of osteogenic cells that will later differentiate into osteoblasts, beginning to form bone matrix on the partially degraded structure of the cartilage model.4 This pathway is responsible for the construction of new healthy bone on the basis of a cartilage model.
Endochondral growth and ossification are affected by various factors, such as biologic parameters, intrinsic genetic predisposition, and systemic chemical agents. In addition, epigenetic mechanical factors have been found to be interacting factors. In areas of intermittent high shear stress, growth may be accelerated, whereas in areas of intermittent high hydrostatic pressure, it may be decelerated, and these latter areas may remain cartilaginous. The articular cartilage or growth plates are examples of this. Today, models are available that allow for calculation of a specific growth rate for each region of cartilage as well as estimation of overall longitudinal growth, taking biologic and mechanobiologic influences into account.5
In the long bones of the skeleton, endochondral ossification spreads outward in both directions from the center of the bone. As the ossification process nears the edges of the cartilage model, chondrocytes proliferate and become hypertrophic, pushing out the cartilaginous end of the bone, forming an area that is termed epiphyseal growth plate, resulting in two centers of endochondral ossification. The primary center lies
in the diaphysis, where chondrocytes replicate and form trabeculae, which are successively replaced by mineralized bone. The perichondrium layer surrounding the former cartilage forms the periosteum, leaving a small foramen for the nutrient artery in the diaphysis. The secondary center of ossification appears at the epiphysis, the region yet to be converted into mineralized bone. Growth plates were found to consist of three layers of chondrocytes during their process of maturation. This area has also been termed the zone of maturation and consists of a region of chondrocyte proliferation, a region of mature chondrocytes, and a region of hypertrophic chondrocytes.6 Apart from the distal femur and the proximal tibia, these secondary ossification centers appear around or after birth.7 With the secured blood supply via epiphyseal arteries, osteogenic cells will later invade the epiphysis and deposit osteoblasts and osteoclasts that will build bone. The process of endochondral ossification occurs in both directions, initiated from the center of long bones. The only exceptions to this are digits and ribs. In case of the formation of ribs and digits, ossification is not directly correlated with the invasion of blood vessels. For example, in ribs, new bone is separated from the perichondrium by a layer of mineralized cartilage; therefore, it cannot be classified as intramembranous ossification. Moreover, there is no preexistence of cartilage as seen in a process of endochondral ossification arising from an epiphyseal growth plate,8 even though text books still describe two to four centers of ossification in the development of ribs.9 The exact pathway remains unknown.
in the diaphysis, where chondrocytes replicate and form trabeculae, which are successively replaced by mineralized bone. The perichondrium layer surrounding the former cartilage forms the periosteum, leaving a small foramen for the nutrient artery in the diaphysis. The secondary center of ossification appears at the epiphysis, the region yet to be converted into mineralized bone. Growth plates were found to consist of three layers of chondrocytes during their process of maturation. This area has also been termed the zone of maturation and consists of a region of chondrocyte proliferation, a region of mature chondrocytes, and a region of hypertrophic chondrocytes.6 Apart from the distal femur and the proximal tibia, these secondary ossification centers appear around or after birth.7 With the secured blood supply via epiphyseal arteries, osteogenic cells will later invade the epiphysis and deposit osteoblasts and osteoclasts that will build bone. The process of endochondral ossification occurs in both directions, initiated from the center of long bones. The only exceptions to this are digits and ribs. In case of the formation of ribs and digits, ossification is not directly correlated with the invasion of blood vessels. For example, in ribs, new bone is separated from the perichondrium by a layer of mineralized cartilage; therefore, it cannot be classified as intramembranous ossification. Moreover, there is no preexistence of cartilage as seen in a process of endochondral ossification arising from an epiphyseal growth plate,8 even though text books still describe two to four centers of ossification in the development of ribs.9 The exact pathway remains unknown.
In the phalanges, the process of ossification is known to take a different course, too. Here, two centers of ossification are described. The primary center of ossification is placed in the diaphysis of the phalanx and ossification begins in the prenatal period. However, only one physis can be seen, located at the base of the phalanges. At the approximate age of 14 to 18 years, all of the epiphyses unite and form the digits.
BONE HEALING
Bone has the capability to repair itself following a fracture. During this process, both pathways to create new bone—intramembranous and endochondral ossification—are applied. However, both require the presence of mesenchymal cells that migrate to the wound site and differentiate into chondrocytes and osteoblasts in response to increased levels of growth factors and cytokines.10 In nonrigid fractures, that is, fractures that retain a certain amount of movement (for instance those immobilized with a cast), the process of endochondral formation of bone will be triggered.
Immediately after the fracture occurs, the physiologic process of wound healing begins and a hematoma is formed. Within the inflammatory phase, the cell debris undergoes phagocytosis and is removed. Blood factors are released and cause the migration and proliferation of fibroblasts, which build granulation tissue.11 This process is termed the reactive phase in the course of fracture healing. Several days after the fracture, a reparative phase is initiated. Periosteal cells proximal to the fracture gap replicate together with the fibroblasts and differentiate into chondroblasts, which form a hyaline cartilage template. The periosteal cells distal to the fracture gap differentiate into osteoblasts that form woven bone until the hyaline cartilage and the woven bone interconnect and form a heterogeneous mass. This mass is called soft callus.
During the next phase of fracture healing, the bone is remodeled using the pathway of endochondral ossification. The callus is invaded by microvessels and osteoblasts, which form new lamellar bone within the earlier mineralized matrix of hyaline cartilage and woven bone.12 This new “hard callus” consists of trabecular bone, already restoring most of the bone’s original strength. In a final phase, this trabecular bone is resorbed by osteoclasts, leaving space for osteoblasts to deposit compact bone within the resorption pit. In time, the callus is remodeled into a state resembling the bone’s original shape and strength. This repair process is fundamentally affected by mechanical loading forces and by the geometric configuration of the fracture fragments, and its finalization may take up to 3 to 5 years.11,13
In comparison, highly stabilized (i.e., rigid) fractures (e.g., those treated with open reduction and stabilization with a metal plate or screws) heal by intramembranous ossification. Here, mesenchymal cells present at the site of injury differentiate directly into osteoblasts to form an osteoid that is later transformed into new bone. The exact pathway of how cells can interpret and respond to changes in the local mechanical environment still remains unclear.14
FORMATION OF A LONG BONE
As pointed out earlier, the long bones of the skeleton are formed on the basis of a cartilaginous model that is created during fetal development. Under the influence of vascular invasion, mesenchymal cells differentiate into osteoclasts and osteoblasts, which in the process of endochondral ossification form an osteoid matrix, from which trabecular bone and, later, mineralized bone is formed. These first centers of ossification appear in the diaphysis of long bones (Fig. 37.1). A perichondral layer of osteogenic cells encircles the cartilaginous model to ensure endochondral growth. Vascular supply is secured through nutrient arteries that invade the long bone via the nutrient foramen, a small opening in the diaphysis, allowing for the migration of osteogenic cells to the primary ossification center. By appositional growth of osteoblasts, longitudinal growth is achieved.
At specific times in the development of each long bone, two secondary centers of ossification appear—the growth plates on either end of the long bone. The growth plate, also known as epiphyseal plate, is an area where formation of new bone is possible. It is located at each end of long bones. Secondary ossification centers mostly occur after birth, with the exception of the growth plate of the distal femur and the proximal tibia, which develop during the perinatal period. From these centers of ossification, the end of each long bone is enlarged by centrifugal growth and allows the process of ossification to spread rapidly from the ossification centers. However, the rate of appositional growth from the secondary center is much slower than that of the primary growth plate. This development of new bone continues until closure of the growth plate at skeletal maturity.
ANATOMY OF THE GROWTH PLATE
As it is well known, a long bone is divided into its proximal epiphysis, proximal metaphysis, diaphysis (shaft), distal metaphysis, and an epiphysis at the distal end. The growth plate
is found in the area of the metaphysis and holds the area of endochondral ossification. As we go from the epiphysis to the diaphysis, there first is an area of articular cartilage forming a cap onto the epiphysis (Fig. 37.2). Especially in long bones such as the femur, where the proximal epiphysis is part of the hip joint, this articular surface is essential for allowing movement in the joint. Therefore, the articular cartilage in this area will be required to transmit weight and will function as a socalled pressure epiphysis. Underneath the articular cartilage, the secondary ossification center of the epiphysis is located. It is supplied by the epiphyseal artery, which enables continuous immigration of osteogenic cells.
is found in the area of the metaphysis and holds the area of endochondral ossification. As we go from the epiphysis to the diaphysis, there first is an area of articular cartilage forming a cap onto the epiphysis (Fig. 37.2). Especially in long bones such as the femur, where the proximal epiphysis is part of the hip joint, this articular surface is essential for allowing movement in the joint. Therefore, the articular cartilage in this area will be required to transmit weight and will function as a socalled pressure epiphysis. Underneath the articular cartilage, the secondary ossification center of the epiphysis is located. It is supplied by the epiphyseal artery, which enables continuous immigration of osteogenic cells.
![]() Figure 37.1. Formation of a long bone: The first centers for ossification appear in the diaphysis of long bones. By appositional growth of osteoblasts, longitudinal growth is achieved. |
The growth plate can be anatomically divided into three types of tissue components: a cartilaginous component (epiphyseal plate), an ossific component (metaphysis), and a fibrous component surrounding the periphery of the plate. The cartilaginous part, which is composed of four different histologic zones, holds the essential stages during which new bone is created. This area is encircled by a fibrous component made up of the groove of Ranvier and the perichondral ring of LaCroix. Adjacent to the cartilage, we find a bony metaphysis.
During childhood, the growth plate consists of connecting cartilage, enabling the long bone to grow in length and girth. At adulthood, the components terminate growth and the growth plate completely ossifies into solid bone.
The Cartilaginous Component
The cartilaginous component of the growth plate is divided histologically into four zones, described as the resting zone, the proliferative zone, the hypertrophic zone, and the zone of provisional calcification (Fig. 37.3).
Located immediately below the secondary center of ossification, there is a zone of undifferentiated or resting cartilage cells, named the reserve zone. This zone contains irregularly scattered cartilage cells with a high lipid content, suggesting
a nutrient storage potential. These cells act as precursor cells for the developing cartilage cells in the next zone. Injury to this zone is deleterious because it often results in a partial or complete arrest of growth. Adjacent to the reserve zone, there is the zone of proliferating cartilage. This zone comprises about half of the overall height of the physis. Although chondrocytes still appear rather flat in this area, they already start to proliferate. At the base of these columns, mitotic activity is found. Cells rapidly divide and become organized into columns, laying down a cartilage extracellular matrix that will later serve as a scaffold for future osteoblasts, which in the future allow the process of ossification. The entire growth in length of the long bone occurs at this location. In the zone of maturation or hypertrophic zone, which follows, cells are still organized in columns, but they enlarge to 5 to 10 times the size of the proliferating zone.
a nutrient storage potential. These cells act as precursor cells for the developing cartilage cells in the next zone. Injury to this zone is deleterious because it often results in a partial or complete arrest of growth. Adjacent to the reserve zone, there is the zone of proliferating cartilage. This zone comprises about half of the overall height of the physis. Although chondrocytes still appear rather flat in this area, they already start to proliferate. At the base of these columns, mitotic activity is found. Cells rapidly divide and become organized into columns, laying down a cartilage extracellular matrix that will later serve as a scaffold for future osteoblasts, which in the future allow the process of ossification. The entire growth in length of the long bone occurs at this location. In the zone of maturation or hypertrophic zone, which follows, cells are still organized in columns, but they enlarge to 5 to 10 times the size of the proliferating zone.
In this layer, there is no active growth and the chondrocytes begin to terminally differentiate, resulting in cell death, triggering blood vessel invasion and bone formation.
As the chondrocytes become hypertrophic, the extracellular matrix, consisting of collagens and proteoglycans, is diminished. This explains why the hypertrophic zone is the weakest: It lacks both collagen and calcified tissue. Therefore, most physeal separations and fractures occur through this layer because it is less resistant to stress than the other parts of the growth plate.
As endochondral ossification depends on the invasion of blood vessels and matrix remodeling, blood vessels enter the partially dismantled scaffold in the zone of provisional calcification. This process allows osteogenic progenitor cells to invade and deposit trabecular bone. The remodeling process causes variations in calcium and phosphate levels in the matrix at the junction of cartilage and bone. The variations in ion concentrations, matrix degradation and mineralization, and influx of new cell types all regulate the equilibrium of cartilage degradation to bone formation.
The Fibrous Structure
Surrounding the periphery of the growth plate lays a wedge-shaped groove of cells, called the groove of Ranvier,15 and a ring of fibrous tissue, the perichondral ring of LaCroix.16
The groove of Ranvier contains cells, which are active in division and contribute in the increase of the width of the growth plate. The structure of the perichondral ring of LaCroix is a fibrous collagenous network that is continuous with the fibrous portion of the groove of Ranvier and the periosteum of the metaphysis. Its function is to mechanically support and stabilize the bone-cartilage junction of the growth plate.17,18,19,20
The groove of Ranvier contains cells, which are active in division and contribute in the increase of the width of the growth plate. The structure of the perichondral ring of LaCroix is a fibrous collagenous network that is continuous with the fibrous portion of the groove of Ranvier and the periosteum of the metaphysis. Its function is to mechanically support and stabilize the bone-cartilage junction of the growth plate.17,18,19,20
The Metaphysis
The metaphysis holds the function of removal of the cartilaginous matrix and its replacement with bone. Its beginning is in the last intact transverse septum of each cartilaginous cell column. Lysosomal enzymes remove the last transverse septum and allow for endothelial and perivascular cells to invade. Between capillaries and osteoblasts that line the calcified bars, osteopro-genitor cells start the process of ossification. This area of only little or no bone formation is termed the primary spongiosa.21 Using the cartilaginous scaffold, osteoblasts progressively form bone, creating the secondary spongiosa. The initial fiber bone is soon replaced by lamellar bone by the process of synchronized osteoclastic bone resorption and osteoblastic bone formation.
Vascular Supply of the Growth Plate
The growth plate and the epiphyseal region are supplied by three vascular systems: the epiphyseal, metaphyseal, and perichondral blood supply.1 The epiphyseal artery mostly secures vascular supply of the epiphysis. It divides into small branches forming a network of vessels, which enter the epiphysis from different sides. Each small branch spreads out in a tree-like fashion and supplies 4 to 10 cell columns. Therefore, the proliferative zone is well perfused to allow for cell proliferation and division.22
In the past, an existence of transphyseal vessels was uncertain and subject to intense controversy. However, recent studies using modern imaging techniques have revealed blood vessels that cross the growth plate.23
The metaphysis is richly supplied with arterial blood from the nutrient artery as well as from the metaphyseal arteries
arising from the ascending cervical arteries. About 80% of the metaphyseal vessels derive from the nutrient artery, which divides into several branches that supply the central region of the metaphysis.
arising from the ascending cervical arteries. About 80% of the metaphyseal vessels derive from the nutrient artery, which divides into several branches that supply the central region of the metaphysis.
THE INFLUENCE OF RECONSTRUCTIVE SURGERY AROUND THE KNEE ON THE DISTAL FEMORAL GROWTH PLATE
Participation of children and adolescents in sports is on the rise, with young athletes training year-round and specializing at earlier ages, predisposing them to sports-related injuries. Once thought to be rare, injuries of the anterior cruciate ligament (ACL) and medial patellofemoral ligament (MPFL) are seen with a greater frequency in the pediatric population. Numerous preventive training programs have been developed but are yet to be established among the society.26 Even though ACL injuries are among the most extensively researched orthopedic injuries and treatment options have evolved, there are still many controversies surrounding the appropriate treatment of these injuries in children and adolescents.
Anterior Cruciate Ligament Injury
Today, there seems to be a consensus among pediatric orthopedic surgeons that reconstructive surgery after a complete tear of the ACL or the MPFL yields a favorable outcome for pediatric and adolescent patients.27,28,29,30,31,32,33,34 Although conservative management is an appealing option given the increased potential of regeneration in children, clinical results after nonoperative treatment have not been satisfactory.35,36,37,38 In cases of partial tears of the ACL, nonreconstructive management may be successful in younger children and adolescents.30,34
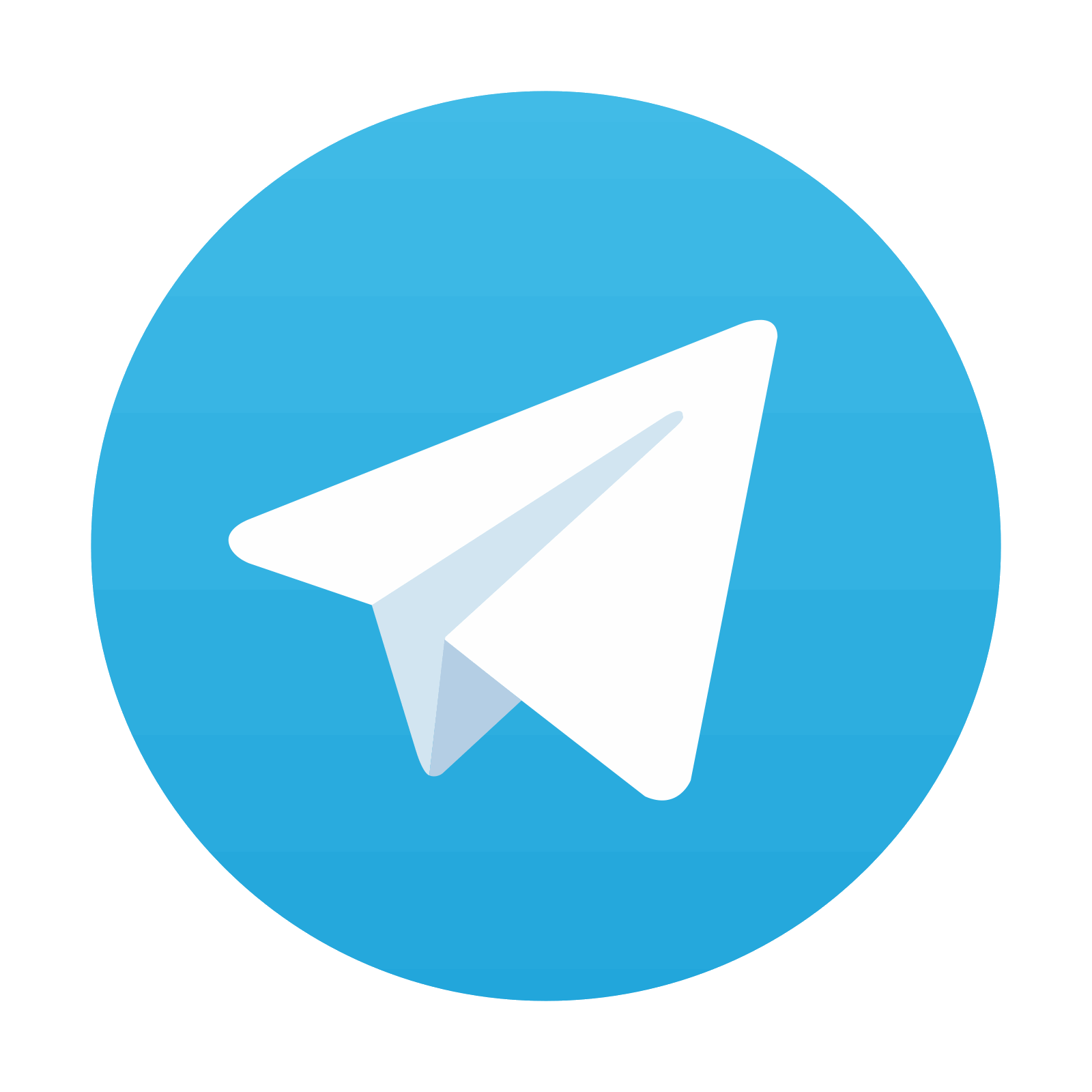
Stay updated, free articles. Join our Telegram channel
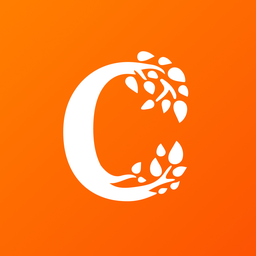
Full access? Get Clinical Tree
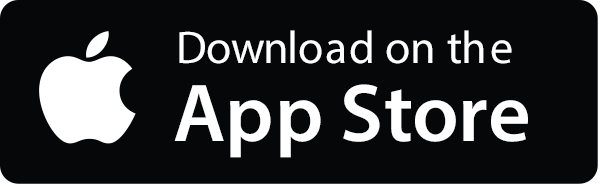
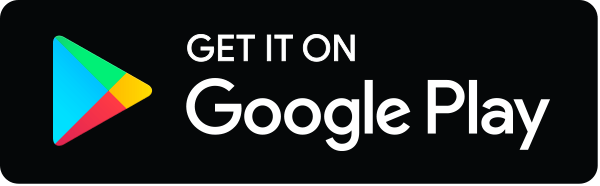