Introduction
Although a reinvigorated global vaccine development effort gives legitimate reason for cautious optimism, it is a sad reality that in 2019 the best introduction to a discussion on vaccines for group A Streptococcus (GAS) comes from 1967, by Professor Gene Stollerman:
… one might have expected that the vast amount of biochemical and immunologic information about this organism would have led to the successful induction of artificial immunity in man against one of his major pathogens. The rewards for such an achievement remain great: the eradication of rheumatic fever and perhaps one major form of glomerulonephritis. The prospect of attaining the same goals by chemotherapy alone is limited … Why, then, have all but a few laboratories abandoned the direct pursuit of so worthy an objective? And what are the prospects for success for those who persist in this tantalizing search?
By any of its multiple names: the anachronistic Streptococcus haemolyticus ; the historical Lancefield Group A β-hemolytic Streptococcus ; the microbiological Streptococcus pyogenes ; or simply “Strep A,” the scourge of GAS diseases has proven to be a remarkably potent and persistent problem. The global burden of GAS diseases spans mild to severe, local and systemic, acute and chronic, infectious and noninfectious syndromes. Although the distribution of GAS diseases has changed with development and control efforts including antibiotics, and the greatest burden continues to fall upon low- and middle-income countries, morbidity and mortality from GAS diseases occur at all ages and in every social and geographic setting. ,
Conservative global estimates of GAS diseases are of 615 million incident cases of GAS pharyngitis, 162 million prevalent cases of impetigo, 660,000 incident cases of invasive GAS infection, 470,000 incident cases of acute poststreptococcal glomerulonephritis (APSGN), and 470,000 incident cases of acute rheumatic fever (ARF), and over 33 million prevalent cases of rheumatic heart disease (RHD). GAS is a major contributor to an estimated 61 million annual cases of cellulitis. , Despite retaining universal susceptibility to penicillin, GAS is associated with at least 500,000 annual deaths, including 320,000 from RHD alone. Case-fatality rates are over 25% for the most severe invasive GAS infections. RHD and APSGN are important contributors to chronic noncommunicable disease states including heart failure, stroke, and chronic kidney disease. Pharyngitis and impetigo cause a less conspicuous but still major impact on healthcare utilization, absenteeism, and antibiotic use. , Inappropriate treatment of suspected GAS pharyngitis in an estimated 70% of cases has a direct cost of $200 million (USD) and a difficult-to-estimate indirect cost associated with antimicrobial resistance. , , Despite this evidence of substantial morbidity, mortality, and cost due to GAS diseases, investment in GAS vaccine development has been miniscule and no current candidate vaccine has entered efficacy trials. ,
The promise of vaccination to prevent ARF and RHD was recognized as the causal link with GAS infection was established. Before that, preceding even recognition and classification of the streptococci, there were active and passive vaccination efforts targeting scarlet fever, another GAS syndrome. , Despite the prodigious proliferation of GAS research continuing since Stollerman’s prophetic words above, vaccine development has been impeded by scientific, regulatory, and commercial obstacles and this promise remains unrealized. , , GAS vaccine development is now a stated priority of the World Health Organization (WHO), as the only healthcare intervention with a realistic prospect of achieving a sizable and sustainable reduction in the global burden of all GAS diseases. , The importance of vaccine development for RHD specifically was recognized in the 2018 WHO Global Resolution on Rheumatic Fever and Rheumatic Heart Disease. Only partial effects have been seen with major public investment in strategies for primary and secondary prevention of ARF and RHD, , and the management of patients with invasive GAS infections regularly stretches the capacities of even the most well-resourced high-technology hospitals. , There is now a coordinated global effort gathering momentum to overcome roadblocks to GAS vaccine development and carry candidate vaccines forward toward Phase 2b/3 trials to demonstrate vaccine safety and efficacy, initially against pharyngitis and possibly impetigo. ,
Issues in GAS Vaccine Development
Strain Diversity. The pioneering streptococcal microbiologist professor Rebecca Lancefield initially segregated the streptococci pathogenic in humans on the basis of the serologic specificity of capsular polysaccharides. Lancefield group A carbohydrate strains, the group A streptococci, were further characterized according to serologic specificity of surface-expressed M-proteins. From approximately 50 M serotypes, molecular typing of the M-protein hypervariable region has now described more than 220 emm genotypes, which have subsequently been grouped by genetic and functional similarities into smaller emm “clusters.” Although the M serotypes, emm genotypes, and emm clusters are related, they are not fully compatible classification systems. Sequencing of the hypervariable emm region and whole genome sequencing has more recently given insight into even greater levels of complexity and strain diversity. ,
The earliest vaccine studies employed relatively crude preparations of mixtures of killed GAS strains. Mid-20th century research focused on the M-protein as the apparent immunodominant GAS antigen, finding that M-serotype-specific bactericidal immune responses correlated with protection against homologous infection but not against infection with heterologous strains. It is largely unknown whether the conclusions reached by these studies finding type-specific bactericidal responses for some serotypes can be extrapolated across all ∼50 M serotypes, or to the >220 emm genotypes, and the extent to which emm clusters correspond to cross-reactive functional antibody responses.
The initial promise of multivalent vaccines targeting strains belonging to emm types most prevalent in high-income countries has been undermined by evidence of extensive global GAS strain diversity, and the potential for rapid emergence of new strains, especially in the highest-burden settings. , The hope that cluster-based cross-reactivity might address this coverage challenge has been only partially substantiated. An alternative but untested approach that has emerged is the notion that a novel synthetic protein vaccine could exploit conserved structural patterns lying beneath more superficial hypervariability of the most immunogenic M-protein regions. A similar approach has also been discussed for vaccines based on the GAS T-antigen. Other groups have shifted focus away from the M-protein hypervariable region to more conserved elements, or away from the M-protein entirely to other conserved antigens. Conserved “cryptic” epitopes from the C-repeat region of the M-protein that are not highly immunogenic after natural exposure have been developed as vaccine antigens, with promising preclinical and phase I results. A growing list of conserved non-M-protein antigens have also shown promise in preclinical studies, some known for many decades (e.g., the group A carbohydrate, streptolysin O, T-antigen) and others more recently identified by reverse vaccinology methods. ,
Protective immune responses. There is no established correlate of natural or vaccine-induced protection against GAS infection in humans and very few longitudinal data from which putative correlates may be inferred. It is a longstanding and widely propagated tenet that decreasing incidence of GAS pharyngitis with age is related to cumulative immunity following natural infections, although this does not offer lifelong protection and there is a spike in incidence of cellulitis and severe invasive GAS infections later in life. A study by Lancefield reporting that, “In 12 individuals type-specific antibodies against 11 different homologous types following 14 separate infections persisted for 4–32 years,” has been widely referenced as indicative of long-lived type-specific protective immunity. However, the sentence immediately following describes another 13 individuals without a type-specific bactericidal response 1–31 years after known GAS infections, including 3 who previously had a homologous type-specific response soon after infection. In 1970s controlled human infection studies with M1, M3, and M12 GAS strains, baseline serum type-specific bactericidal responses did not clearly correlate with protection against experimental pharyngitis caused by homologous strains applied by swab to the pharynx of healthy adult volunteers. Others have demonstrated that antibody responses targeting the group A carbohydrate correlate with protection against GAS pharyngeal colonization, and in vitro responses against a number of other antigens do increase with age.
Development of methods to measure relevant immune responses is central to vaccine development. For GAS, there are presently no widely available standardized high-throughput methods. Widely used enzyme-linked immunosorbent assays to measure binding antibody suffer from a lack of standardization and reference GAS sera. Work continues on newer opsonophagocytic functional antibody assays to replace the laborious Lancefield serum bactericidal assay, which does not produce results that can be compared between assays or laboratories. , Qualification and validation of these newer assays, with availability of GAS reference serum, will be an important step forward.
Autoimmunity. The specter of autoimmune complications induced by vaccines against GAS has arguably been the greatest obstacle standing in the way of vaccine development. ARF (with subsequent RHD) and APSGN are nonsuppurative immunological complications following GAS infections. The pediatric autoimmune neuropsychiatric disorders associated with streptococcal infections (PANDAS) hypothesis are also discussed in these terms. There have been extensive studies of humoral and cellular cross-reactivity and homology between human proteins and streptococcal antigens, focusing mostly on M-protein but also on non-M antigens including the group A carbohydrate (discussed in detail in Chapter 2 ). Autoreactive T lymphocytes recognizing the N-terminal and midprotein domains of the M-protein have been described in ARF and cloned from the valves of RHD patients. Although many studies have found cross-reactivity and homology, particularly for some M-protein regions and the G1cNAc moiety of the group A carbohydrate, none has conclusively implicated an antigen or antigenic region in the causal pathway of ARF or APSGN in humans. Nonetheless, vaccine developers have been understandably cautious in avoiding the potentially cross-reactive antigens in their candidates.
Animal models. GAS is exquisitely adapted and restricted to its human host. Professor Rebecca Lancefield illustrated this by recounting the difficulty she had experienced in attempts to infect mice, requiring multiple repeated passages, whereas accidental exposure of laboratory assistants to such a strain easily caused infection in humans, even when the strains had not seen a human host in many years. It is possible to passage strains for adaption to some other species (typically mammalian) such as mice, or if nonhuman primates are inoculated with very high doses of bacteria. Still, human host restriction for certain virulence factors may not be overcome, although humanized transgenic models can go some way to addressing this problem. Importantly, even if every animal model was considered together, the diversity of human GAS disease syndromes has not been reproduced by animal models.
History of GAS Vaccines
Recent reviews have suggested that the first clinical trial of a GAS vaccine was in 1923 by Bloomfeld et al. following an earlier finding by the same group that asymptomatic carriage of GAS prevented symptomatic pharyngitis. , However, there is also an important and latterly under-appreciated prehistory provided by publications describing vaccine studies targeting scarlet fever. , From 1833 to 1923, healthy adults and/or child participants in these studies were administered variously processed extracts of blood, serum, mucosal (pus, mucous) or cutaneous lesions (crusts) from scarlet fever cases, with the goal of inducing protective immune responses. The earliest of these studies explicitly hoped to emulate Edward Jenner’s success in protecting against smallpox by inoculation of cowpox ( Variolae vaccinae ), an approach suggested in 1796 by Erasmus Darwin (Charles’ grandfather):
No one could do an act more beneficial to society, or glorious to himself, than by teaching humanity how to inoculate this fatal disease [scarlet fever]; and thus, to deprive it of its malignity. Matter might be taken from the ulcers in the throat, which would probably convey the contagion. Or warm water might be put on the eruption and scraped off again by the edge of a lancet. These experiments could be attended with no danger, and should be tried for the public benefit, and the honor of medical science.
Although these scarlet fever inoculation experiments were of immense value in understanding the pathogenesis of scarlet fever, and more than one study led to brief and limited use of a product as an intended vaccine, none demonstrated sufficiently convincing protection to justify continued development.
In 1923, a heat-killed whole cell vaccine comprising 21 GAS strains isolated from recent cases of acute pharyngitis was administered by subcutaneous injection to female nursing student volunteers. Across all 90 participants, over 4 months there were 3/35 (8.6%) cases of acute pharyngitis in vaccine recipients and 12/55 (21.8%) in those who received the placebo control (VE 61%; RR 0.39, 95% CI 0.12–1.29). There were zero cases of acute pharyngitis in vaccinated or control subjects who had asymptomatic GAS throat carriage at baseline. Considering only noncarriers, 3/18 (16.7%) vaccinated subjects developed pharyngitis compared to 12/39 (30.8%) controls (VE 46%; RR 0.54, 95% CI 0.17–1.69). For “severe” pharyngitis (fever ≥102°F; marked prostration, “violent pharyngeal reaction”), 0/18 of the vaccinated noncarriers were affected compared to 11/39 of the unvaccinated subjects (VE 100%; RR 0.09, 95% CI 0.006–1.47).
Subsequent to the promising study by Bloomfeld et al., a number of killed whole-cell vaccines were studied, and then partially purified M-protein vaccines ( Table 14.1 ), with mixed results and/or unacceptable reactogenicity. During World War II, the US Navy tested a killed whole-cell vaccine (M17 and M19 serotypes) in recruits, with no protective efficacy observed even against M17 and M19—GAS pharyngitis persists as a problem in US military recruits to this day, and penicillin prophylaxis is still used. , Reactogenicity concerns were addressed by development of improved M-protein purification techniques.
Publication Year | Vaccine b | References |
---|---|---|
1923 | Heat-killed whole-cell 21-strains | |
1930 | Heat-killed whole-cell | |
1931 | Heat-killed whole-cell | |
1932 | Heat-killed whole-cell | |
1933–1943 | “Toxin” and tannic acid-precipitated “toxin” | |
1937–1941 | Tannic acid-precipitated “toxin” | |
1946 | Heat-killed or ultraviolet-inactivated whole-cell M17 and M19 | |
1949 | Heat-killed whole-cell M3 and M17 | |
1960 | Partially purified M19 | |
1962 | M5 and M12 cell wall | |
1963 | M14 cell wall | |
1968 | Partially purified M3 protein | |
1969 | Highly purified M12 protein | |
1973 | Highly purified M1 protein | |
1975 | Highly purified M1 protein (mucosal) | |
1978 | Highly purified M3 protein (parenteral and mucosal vaccines) Highly purified M12 protein (parenteral and mucosal vaccines) | |
1979 | Polypeptide fragment M24 protein |
a Table adapted with permission from Steer et al. .
Between 1973 and 1978, three important proof-of-concept human infection (controlled human infection, challenge) studies were published, including 172 participants in total. In each study, parenteral or mucosal M-protein vaccines were administered to healthy volunteers who were subsequently challenged with homologous serotype strains by direct application to the pharynx using a swab. In the initial study of a parenteral highly purified monovalent M1-protein vaccine, protective efficacy against pharyngitis caused by a homologous M1 strain was 89% (95% CI 23–98). This was followed by a study using a mucosal M1 vaccine, with vaccine efficacy of 68% (28–86). Efficacy could not be demonstrated in the underpowered third study testing separate parenteral and mucosal M3 and M12 vaccines against challenge with homologous strains. Across the three studies, colonization (positive throat cultures) was significantly reduced by mucosal but not parenteral vaccination, and vaccination (of any kind) was associated with statistically significant reductions in every symptom and sign of pharyngitis. There were inconsistent quantitative (complement fixation, hemagglutination, radioimmune precipitation) and functional (bactericidal assay) antibody responses in serum and nasal secretions following vaccination and challenge, and none was predictive of protection against pharyngitis or colonization.
Unfortunately, a hiatus in GAS vaccine research and development occurred in the aftermath of a trial by Massell et al. administering between 18 and 33 weekly doses of a partially purified acid extract of M3 protein to 21 siblings of patients with ARF. There were two definite cases and one probable case of ARF subsequently reported in study subjects after infection with heterologous GAS serotypes, with concerns raised in 1969 that the vaccine might be responsible as the rate appeared higher than expected compared to a historical cohort of siblings from the same facility. Although the authors advised that the study design and small number of participants prevented firm conclusions from being made, the US Food and Drug Administration (FDA) responded in 1979 by issuing an embargo against further use of, “uncontrolled, poorly defined preparations presumed to contain antigens that have been demonstrated in earlier studies to produce local and systemic reactions.” Although the 2006 revocation of this ruling suggests the intention was that this would not apply to highly purified well-characterized GAS antigens without demonstrable cross-reactivity with human tissues, the ruling did cast a pall on GAS vaccine research and development, with the practical effect of a ban.
21st Century GAS Vaccine Development
GAS vaccine research and development in the period following revocation of the FDA ruling can most easily be considered according to the various M-protein and non-M-protein targets of candidate vaccines ( Table 14.2 ). Although there has been a steady increase in the number of preclinical vaccines since 2005, there has been a frustrating lack of progress in human clinical trials, with only four products tested in phase 1 and one vaccine reaching phase 2. None has entered efficacy trials, and major vaccine industry players have largely remained on the sidelines.
Candidate Name/Identifier | Stage of Development | Reference | ||
---|---|---|---|---|
Preclinical | Phase I | Phase II | ||
M-protein: 6-valent N-terminal | X | X | ||
M-protein: 26-valent N-terminal | X | X | X | |
M-protein: 30-valent N-terminal | X | X | ||
M-related proteins | X | , | ||
M-protein: minimal epitope J8 | X | X | ||
P∗17: synthetic derivative of J8 | X | |||
M-protein: minimal epitope J14/p145 | X | |||
M-protein: five J14 variants (SV1) | X | |||
M-protein: divalent N-terminal M1 + M12, with J14 | X | |||
M-protein: 10-valent N-terminal, expressed by live Lactococcus lactis | X | |||
M-protein: C-repeat epitope (StreptInCor) | X | |||
M-protein: C-repeat epitopes | X | |||
Three conserved antigens (Combo): SLO, SpyAD, SpyCEP (currently in development with fourth antigen—group A carbohydrate) | X | |||
Five conserved antigens (Combo5): arginine deiminase, C5a peptidase, streptolysin O, SpyCEP, trigger factor | X | , | ||
Seven conserved antigens (Spy7): C5a peptidase, oligopeptide-binding protein, putative pullulanase, nucleoside-binding protein, hypothetical membrane associated protein, cell surface protein, SpyAD | X | , | ||
Group A carbohydrate (GAC) | X | |||
Group A carbohydrate (GAC) defective for GlcNAc side-chain | X | |||
C5a peptidase (SCPA) | X | |||
Sortase A (SrtA) + C5a peptidase (SCPA) | X | |||
Fibronectin-binding protein (FBP) | X | , | ||
Streptococcal protective antigen | X | |||
Serum opacity factor (SOF) | X | |||
Streptococcal pyrogenic exotoxin A/B/C (SpeA, SpeB, SpeC) | X | |||
Streptococcal pyrogenic exotoxin (SpeAB) fusion protein | X | |||
Streptococcal pili (T-antigen) | X | , | ||
Serine protease (SpyCEP or S2 subunit) | X | , | ||
Nine common antigens | X |
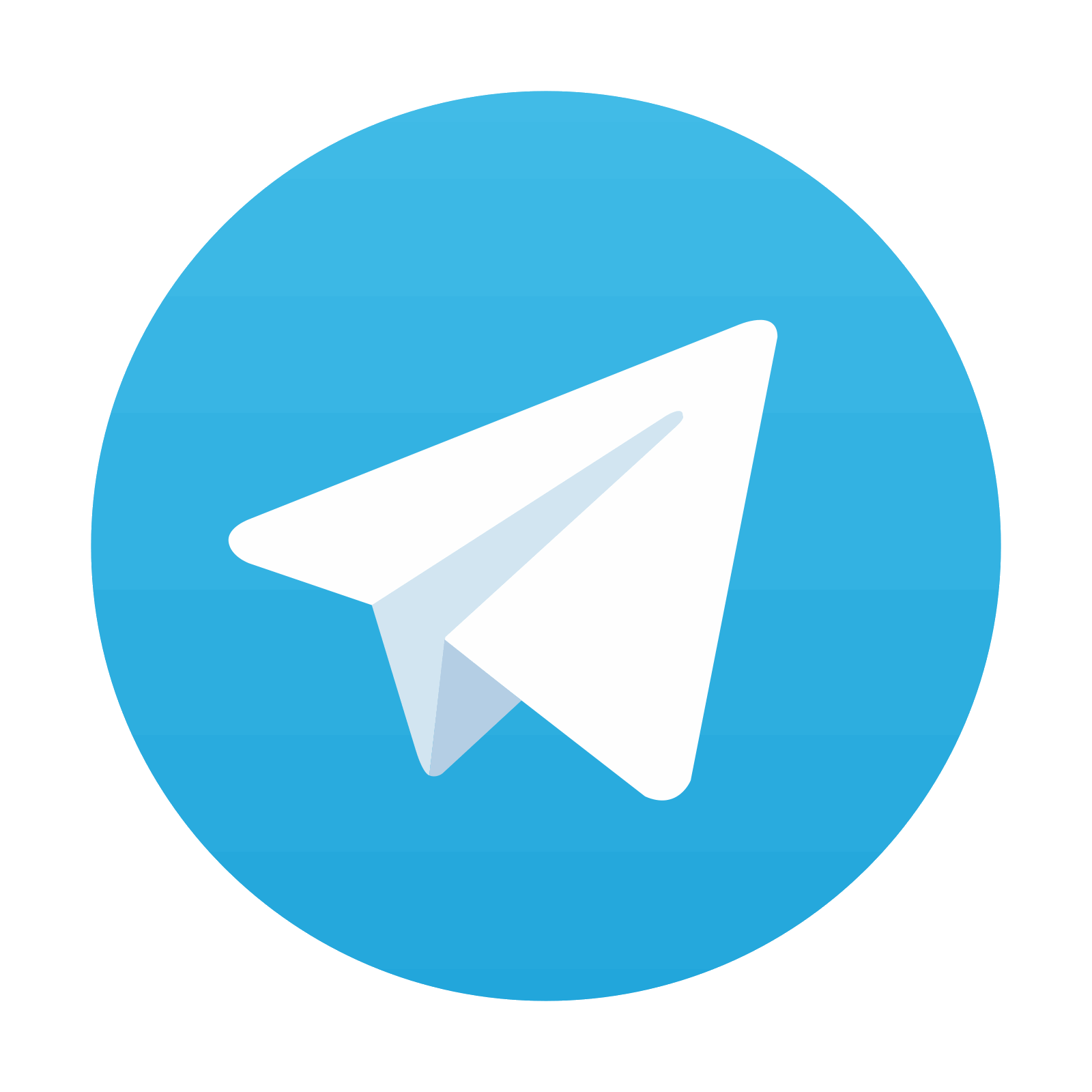
Stay updated, free articles. Join our Telegram channel
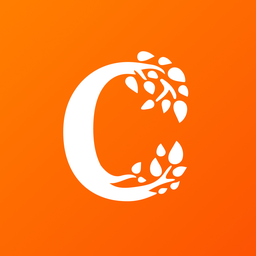
Full access? Get Clinical Tree
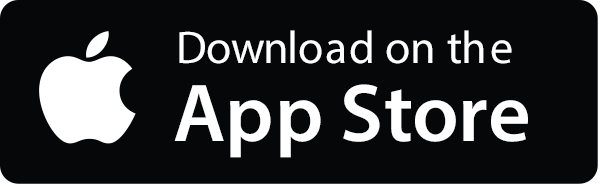
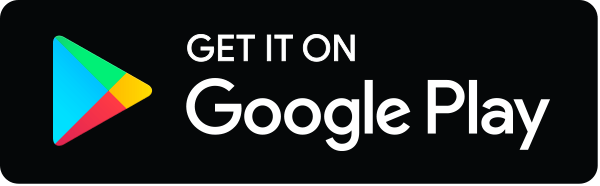
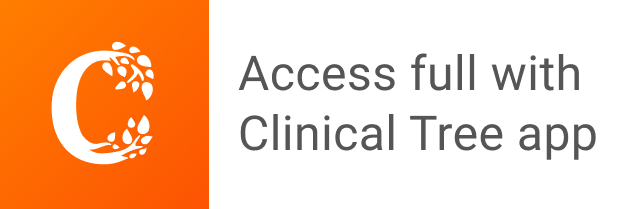