Chapter Outline
Properties of the Immature Skeleton 1199
Care of the Multiply Injured Child 1211
Open Fractures 1214
Compartment Syndrome 1217
Vascular Injuries 1219
Casts 1220
Child Abuse 1222
Summary 1223
Skeletal injuries are common in children, with an estimated 40% of boys and 25% of girls sustaining a fracture by 16 years of age. Because of the properties of the immature skeleton, these injuries have different characteristics, complications, and management than those of similar injuries in adults.
A number of studies have examined the epidemiology of fractures in children. Most studies have shown a male predominance, particularly in adolescence. Fractures in children younger than 18 months are rare and should raise the question of nonaccidental trauma. Combining the data from five large epidemiologic studies reveals fractures of the distal forearm to be the most common fracture in children, accounting for almost 25% of 12,946 fractures. The clavicle is the next most commonly injured site, representing over 8% of all children’s fractures ( Table 31-1 ).
Parameter | Epidemiologic Study | Total (%) * | ||||
---|---|---|---|---|---|---|
A | B | C | D | E | ||
Total fractures in series | 923 | 2040 | 410 | 291 | 8682 | 12,346 (100) |
Anatomic Site | ||||||
Clavicle | 58 | 222 | 55 | 45 | 703 | 1083 (8.8) |
Humerus (proximal end and shaft) | 18 | 81 | 14 | 13 | 126 (1.0) | |
Distal humerus | 71 | 158 | 68 | 287 | 584 (4.7) | |
Radial neck | 25 | 45 | 1 | 104 | 175 | 350 (2.8) |
Radius, ulna (shafts) | 60 | 108 | 23 | 39 | 295 | 525 (4.3) |
Distal radius, ulna | 330 | 755 | 81 | 80 | 1971 | 3217 (26.1) |
Hand | 136 | 494 | 88 | 718 (5.8) | ||
Femur | 18 | 87 | 27 | 13 | 145 | 290 (2.3) |
Tibia, fibula (shafts) | 40 | 256 | 19 | 10 | 434 | 759 (6.1) |
Ankle | 37 | 61 | 28 | 14 | 478 | 618 (5.0) |
Foot | 71 | 172 | 28 | 271 (2.2) |
* Because not all fractures are listed, the percentages of fractures do not total 100%.
Properties of the Immature Skeleton
The immature skeleton has an increased adaptation to stress prior to failure, thicker periosteum, potential to remodel, shorter healing time, and a physis.
Plastic Deformation
A few studies have compared the mechanical properties of bone in children and bone in adults. Immature bone is weaker in bending strength but absorbs more energy before fracture. This is a result of the ability of immature bone to undergo plastic (permanent) deformation ( Fig. 31-1 ). Although plastic deformation has been described in adults, it is much more common in children. Plastic deformation is most common in the forearm, particularly the ulna, especially after isolated radial head dislocation; however, it has been noted in the femur as well. *

* References .
Although bone in young children may remodel after plastic deformation, most authors recommend reduction of plastic deformation of the forearm if there is more than 20 degrees of angulation or the child is older than 4 years and has a clinically evident deformity or limitation of pronation and supination. Sanders and Heckman were able to reduce an average of 85% of the angulation noted at the time of injury using a fulcrum to apply a steady force at the apex of the deformity for several minutes with the patient under general anesthesia.Fractures
Buckle (Torus) Fractures
Buckle fractures, also called torus fractures because of their resemblance to the base of an architectural column, most commonly occur at the transition between the metaphyseal woven bone and lamellar bone of the diaphyseal cortex ( Fig. 31-2 ). Buckle fractures represent a spectrum of injuries from mild plastic deformation of one area of the cortex to complete fractures with a buckled appearance.

It is not uncommon for torus fractures to be diagnosed several days or even weeks after injury because the pain and swelling may be attributed to a sprain. Although most torus fractures can be managed successfully with minimal symptomatic treatment, it is important to identify minimally displaced complete fractures that have a buckled appearance because they are potentially unstable and may displace if not managed with a well-molded cast ( Fig. 31-3 ). Although such late displacement is usually mild and remodels with no sequelae, parents are often upset when the fracture is more displaced when the cast is removed than at the time of injury.

Greenstick Fractures
Greenstick fractures are unique to children because immature bone is more flexible and has a thicker periosteum than mature adult bone. In a greenstick fracture, the cortex in tension fractures completely whereas the cortex and periosteum in compression remain intact but frequently undergo plastic deformation. It has been said that it is necessary to complete the fracture on the intact compression side of greenstick fractures, but this has not been my experience. We believe it is necessary only to achieve an acceptable reduction of a greenstick fracture. To reduce a greenstick fracture, it is usually necessary to unlock the impacted fragments on the tension side by initially exaggerating the deformity and then applying traction and a reducing force. In my experience, whether the fracture is completed during the exaggeration of the deformity has not been important. Because of the intact cortex and periosteum, greenstick fractures are usually stable after reduction ( Fig. 31-4 ); however, there is an increased likelihood of refracture. I usually immobilize these fractures for a full 6 weeks and warn the parents that although they are usually simple to reduce, they are more likely to refracture.

Remodeling and Overgrowth
Not only do children’s fractures heal more rapidly than those in adults, but once healed, they have the potential to remodel residual deformity ( Fig. 31-5 ). Factors that affect the remodeling potential of a deformity include the amount of growth remaining and the plane of the deformity in relation to adjacent joints. †

† References .
The single most important factor determining how much growth will contribute to the remodeling potential of a fracture is the patient’s skeletal age. Other factors include the deformity’s proximity to the physis and growth potential of the particular physis. For example, because 80% of the growth of the proximal humerus comes from the proximal physis, deformity associated with proximal humeral fractures is much more likely to remodel than deformity associated with distal humeral fractures.Wolff’s law states that bone remodels according to the stress placed across it. It follows that posttraumatic deformity in the plane of motion of a joint will have greater potential to remodel than deformity not in the plane of motion. This is demonstrated with fractures of the femoral shaft, which remodel a large amount of sagittal plane deformity, a lesser amount of coronal deformity, and little or no rotational deformity.
Another consideration in the management of children’s fractures is the potential for the accelerated growth of an injured limb. Clinically, this is most frequently seen in diaphyseal femoral fractures. It has long been recognized that fractures of the femoral shaft will spontaneously correct shortening of up to 2 cm. ‡
‡ References .
It has been hypothesized that this overgrowth is a result of hyperemia associated with the fracture. However, evidence casts some doubt on this theory. First, fractures of the radius do not demonstrate this propensity for overgrowth. Second, efforts to stimulate blood flow by periosteal stripping do not result in permanent growth increases. Finally, anatomic reduction of femoral shaft fractures treated operatively has not resulted in significant overgrowth. Thus there may exist some other yet to be determined factor that predisposes an injured extremity to return to its normal, preinjury length.Physeal Injuries
Physeal injuries represent 15% to 30% of all fractures in children. The incidence varies with age and has been reported to peak in adolescence. Physeal injuries involving the phalanges have been reported to account for over 30% of all physeal fractures. Fortunately, although physeal injuries are common, growth deformity is rare, occurring in only 1% to 10% of all physeal injuries.
Although problems arising from physeal injury are rare, they are often predictable and occasionally preventable. A basic understanding of the anatomy and physiology of the physis and its response to injury is necessary to manage injuries to the growth plate effectively.
Physeal Anatomy
It is important to distinguish the physis (also referred to as the epiphyseal plate, epiphyseal growth plate, or epiphyseal cartilage) from the epiphysis, or secondary ossification center. The physis is connected to the epiphysis and metaphysis by the zone of Ranvier and the perichondral ring of LaCroix ( Fig. 31-6 ). The zone of Ranvier is a wedge-shaped group of germinal cells that is continuous with the physis and contributes to latitudinal, or circumferential, growth of the physis. The zone of Ranvier consists of three cell types—osteoblasts, chondrocytes, and fibroblasts. Osteoblasts form the bony portion of the perichondral ring at the metaphysis, chondrocytes contribute to latitudinal growth, and fibroblasts circumscribe the zone and anchor it to perichondrium above and below the growth plate. The perichondral ring of LaCroix is a fibrous structure that is continuous with the fibroblasts of the zone of Ranvier and the periosteum of the metaphysis. It provides strong mechanical support for the bone-cartilage junction of the growth plate.

The physis consists of chondrocytes in an extracellular matrix. Both the chondrocytes and matrix are preferentially oriented along the longitudinal axis of long bones. The physis has traditionally been divided into four zones—the resting or germinal zone, proliferative zone, zone of hypertrophy, and zone of enchondral ossification, which is continuous with the metaphysis (see Fig. 31-6 ). The first two zones have an abundant extracellular matrix and, consequently, a great deal of mechanical integrity, particularly in response to shear forces. The third layer, the hypertrophic zone, contains scant extracellular matrix and is weaker. On the metaphyseal side of the hypertrophic zone there is an area of provisional calcification leading to the zone of enchondral ossification. The calcification in these areas provides additional resistance to shear. Thus the area of the hypertrophic zone just above the area of provisional calcification is the weakest area of the physis, and it is here that most injuries to the physis occur. The fact that the cleavage plane through the physis is through the hypertrophic zone implies that after most injuries, the germinal layer of the physis remains intact and attached to the epiphysis. Thus, provided that there is no insult to the blood supply of the germinal layer or development of a bony bridge across the injured physis, normal growth should resume after an injury.
Two types of epiphyseal vascularization have been identified in a primate model ( Fig. 31-7 ). Type A epiphyses are almost entirely covered by articular cartilage. In these epiphyses, the blood supply enters the periphery after traversing the perichondrium. Consequently, the blood supply is vulnerable to damage if the epiphysis is separated from the metaphysis. The proximal femur and proximal radius are the only two type A epiphyses. Type B epiphyses are only partially covered by articular cartilage. Their blood supply enters from the epiphyseal side and is protected from vascular injury during separation.

Harris Growth Arrest Lines
Harris is credited with the first radiographic observation of bony striations in the metaphysis of long bones. These Harris growth arrest lines are transversely oriented condensations of normal bone and are thought to represent slowing or cessation of growth corresponding to times of illness, injury, or healing. They may be present in a single bone after an isolated traumatic injury or in all long bones after a significant systemic illness. When present after a physeal injury, they serve as an effective representation of the health of the physis. If the growth arrest line is transverse and parallel to the physis, the physis can be assumed to be growing normally. If there has been a partial injury to the physis, the growth arrest line will be asymmetric. There will be no growth arrest line if there has been no growth following a total physeal injury ( Fig. 31-8 ). Harris growth arrest lines may also be seen on magnetic resonance imaging (MRI) scans.

Classification of Physeal Injuries
Over the years, a number of classification systems for physeal injuries have been described, including those by Foucher, Poland, Aitken, and Ogden. However, the most widely used system is that of Salter and Harris ( Fig. 31-9 ).

A Salter-Harris type I injury is a separation of the epiphysis from the metaphysis that occurs entirely through the physis. It is rare and is seen most frequently in infants or in pathologic fractures, such as those secondary to rickets or scurvy. Because the germinal layer remains with the epiphysis, growth is not disturbed unless the blood supply is interrupted, as frequently occurs with traumatic separation of the proximal femoral epiphysis.
In a Salter-Harris type II injury, the fracture extends along the hypertrophic zone of the physis, and at some point exits through the metaphysis. The epiphyseal fragment contains the entire germinal layer as well as a metaphyseal fragment of varying size. This fragment is known as the Thurston Holland sign. The periosteum on the side of the metaphyseal fragment is intact and provides stability once the fracture is reduced. Growth disturbance is rare because the germinal layer remains intact.
In a Salter-Harris type III injury, the fracture extends along the hypertrophic zone until it exits through the epiphysis. Thus, by definition, type III fractures cross the germinal layer and are usually intraarticular. Consequently, if displaced, they require an anatomic reduction, which may need to be achieved open.
Salter-Harris type IV injuries extend from the metaphysis across the physis and into the epiphysis. Thus the fracture crosses the germinal layer of the physis and usually extends into the joint. As in type III injuries, it is important to achieve an anatomic reduction to prevent osseous bridging across the physis and restore the articular surface.
A Salter-Harris type V injury is a crushing injury to the physis from a pure compression force. It is so rare that Peterson and Burkhart have questioned whether such an injury can occur. Those who have reported Salter-Harris type V injuries have noted a poor prognosis, with an almost universal growth disturbance.
Although the Salter-Harris classification of physeal fractures is the most widely used system, there are a few physeal injuries that do not fit into this classification scheme. The first is an injury to the perichondral ring. Salter’s colleague Mercer Rang, termed this a type VI physeal injury ( Fig. 31-10 ). (This injury is also included in Ogden’s classification.) Basing his system on a review of 951 fractures, Peterson proposed a new classification scheme ( Fig. 31-11 ). Although this classification system has many similarities to that of Salter-Harris, its important addition is the Peterson type I fracture, a transverse fracture of the metaphysis with extension longitudinally into the physis. Clinically this fracture is commonly seen in the distal radius. Peterson also described a type VI injury, which is an open injury associated with loss of the physis.


Treatment of Physeal Injuries
In general, the principles involved in the treatment of physeal injuries are the same as those involved in the treatment of all fractures, although there are a few important caveats. As with all traumatic injuries, before an injury to the physis is treated, the patient must be thoroughly assessed using the ABCs of trauma (see later, “Care of the Multiply Injured Child”).
Once the child has been stabilized and all life- and limb-threatening injuries identified, a treatment plan can be developed. It is important to remember that physeal fractures can and often do coexist with neurovascular or open injuries. When this occurs, the physeal fracture is treated after appropriate management of the soft tissue injuries.
The goal in treating physeal fractures is to achieve and maintain an acceptable reduction without subjecting the germinal layer of the physis to any further damage. The most subjective of these goals, and perhaps the most important, is determining the limits of an acceptable reduction. A number of factors must be considered when assessing a nonanatomic reduction. These include the amount of residual deformity, location of the injury, age of the patient, and amount of time that has elapsed since the injury. The location of the injury and patient’s age are determining factors in the bone’s remodeling potential. Obviously, more deformity can be accepted if the potential to remodel is high. Rang and Salter recommended accepting any displacement in type I or II injuries after 7 to 10 days, believing that it is safer to perform an osteotomy later than to risk injuring the physis with a traumatic reduction of a physeal fracture that has begun to heal. Interestingly, this recommendation has been accepted and repeated with little clinical or experimental evidence to prove its validity. Egol and colleagues studied the effect of a delay in reduction of Salter-Harris I fractures in rats. There was no evidence that a delay in reduction produced a growth disturbance. Despite this animal study, I believe it is still prudent to avoid reduction in late-presenting type I or II physeal fractures. Because of the intraarticular component, displaced type III and IV injuries must be reduced, regardless of the time that has elapsed since the injury.
Once a physeal fracture has been reduced, the reduction can be maintained with a cast, pins, internal fixation, or some combination of these. Specific recommendations regarding the method and duration of immobilization are discussed in the specific chapters in the text pertaining to each injury.
Complications of Physeal Injuries
Like all fractures, physeal injuries may be complicated by malunion, infection, neurovascular problems, or osteonecrosis. The treatment of these complications is discussed elsewhere in the text in the context of specific injuries.
A complication unique to physeal fractures is growth disturbance. Although sequelae of injury are the most common causes of growth disturbance, it is also seen as a consequence of Blount disease, infection, irradiation, thermal injury, and laser beam exposure. Although physeal injuries represent 15% to 30% of all fractures, growth arrest occurs after only 1% to 10% of physeal fractures. A number of factors affect the likelihood of growth arrest. Comminuted fractures from high-energy injury are more likely to result in physeal arrest. Physeal injuries that cross the germinal layer (Salter-Harris types III and IV injuries) are also more likely to be associated with subsequent growth disturbance. Fortunately, not all patients in whom a physeal arrest develops will require treatment. This is because physeal injuries are most common in adolescents, who often have limited growth remaining and, consequently, limited expected clinical disturbance.
Assessment of Growth Disturbance
Growth disturbance from a physeal fracture is usually evident 2 to 6 months after the injury, but it may not become obvious for up to 1 year. Thus it is important not only to warn parents about this potential problem but to follow patients with physeal fractures long enough to identify growth arrest. Early identification of a traumatic growth disturbance can make its management considerably easier because the treatment can be directed solely toward resolving the arrest, rather than treating both the arrest and an acquired growth deformity. Growth disturbance is usually the result of the development of a bony bridge, or bar, across the physeal cartilage. However, growth disturbance may occur after traumatic injury without the development of a bony bridge, presumably because the injury slows the growth of a portion of the physis rather than stopping it completely. The resulting asymmetric growth can produce clinically significant angular deformity ( Fig. 31-12 ).

The development of a bony bar may create a complete or partial growth disturbance. If the area of the bar is large, it may stop the growth of the entire physis ( Fig. 31-13 ). More often, a bar forms in a portion of the physis and stops growth at that point while the rest of the physis continues to grow. This produces a tethering effect, which may result in shortening, progressive angular deformity, or both ( Figs. 31-14 and 31-15 ).



To treat a physeal bar appropriately, the extent and location of the bar and amount of growth remaining from the physis must be determined. The anatomy of a physeal bar may be delineated using plain radiography, tomography, computed tomography (CT), or MRI. MRI is increasingly used to assess physeal anatomy and has replaced CT as my preferred imaging modality. In particular, fat-suppressed, three-dimensional, spoiled gradient–recalled echo sequences can be obtained and reconstructed to create an accurate three-dimensional model of the physis, including calculation of the percentage of physeal arrest ( Fig. 31-16 ). Partial physeal arrests are usually classified as peripheral (type A) or central (type B or C), depending on their location within the physis ( Fig. 31-17 ). There are two types of central bars. Type B is surrounded by a perimeter of healthy physis. This type of bar may produce a tethering effect that tents the epiphysis and produces a joint deformity. In type C, the bar traverses the entire physis from front to back (or side to side). The physis on both sides of the bar is normal. This pattern is commonly seen with injuries to the medial malleolus.


Once the extent and location of the bar have been defined, the amount of growth remaining from the physis must be determined. This can be accomplished by determining the skeletal age of the patient and using information on growth patterns assembled by Green and Anderson. Skeletal age can be determined by comparing a radiograph of the left hand and wrist with standards in an atlas of skeletal age. It is generally assumed that girls grow until a bone age of 14 years and boys until a bone age of 16 years. Future growth for the distal femur and proximal tibia can be estimated using the graphs initially published by Anderson and colleagues ( Fig. 31-18 ) or by using approximations of yearly physeal growth ( Table 31-2 ).

Location | Yearly Growth (mm) |
---|---|
Proximal femur | 2 |
Distal femur | 9 |
Proximal tibia | 6 |
Distal tibia | 4 |
Proximal humerus | 12 |
Distal radius | 8 |
Treatment of Physeal Arrest
Treatment options for physeal arrests include observation, completion of a partial arrest, or physeal bar resection.
Observation.
If the bar appears to involve the entire physis and there is an acceptable existing limb length inequality or angular deformity and little contralateral growth remaining, observation may be the best option.
Completion.
Completion of a physeal bar may be indicated if there is an acceptable existing angular deformity that might become clinically unacceptable if untreated. With completion of an arrest, the surgeon must evaluate the likelihood of a subsequent limb length inequality. If the likelihood of significant limb length inequality (>20 to 25 mm) is high, a contralateral epiphysiodesis should be performed at the time of completion of the partial arrest.
Resection.
Resection of a physeal bar is indicated for partial arrests with substantial growth remaining. Physeal bar resection was first introduced by Langenskiöld and has been studied in human and animal models. The technique of bar excision involves removing the bone bridging the metaphysis and epiphysis and filling the void created with an interposition material that will prevent reformation of the bony bar. The remaining physis must be undamaged and must be large enough that growth is likely to continue. In addition, there should be a significant amount of growth remaining before skeletal maturity and physiologic physeal closure. Numerous studies have documented that bars involving more than 50% of the physis are unlikely to respond to bar resection. §
§ References .
Recommendations regarding requirements of amount of growth remaining are less uniform, with opinions varying from 1 to 2 years of growth remaining. Clearly, the younger the patient and the more the potential growth from the physis, the greater the benefit of a successful resection.The surgeon must decide whether an osteotomy is necessary to correct an existing angular deformity. Although angular deformities less than 20 degrees have been reported to correct spontaneously after bar resection, this has not been my universal clinical experience. In addition, the Hueter-Volkmann principle indicates that improved alignment may help facilitate more normal growth. I believe that a corrective osteotomy should be considered for any angular deformity judged to be clinically unacceptable.
When a peripheral (type A) bar is resected, the bar should be approached directly and removed under direct vision, with a wide margin of periosteum ( Fig. 31-19 ). The bar should be resected until the cavity is rimmed completely with normal physis. Types B and C bars are approached through a window in the metaphysis or through an osteotomy. Although some have advocated magnification with loupes or a microscope, I have not routinely used these. However, resection of central bars can be facilitated by the use of fluoroscopy, fiberoptic lighting, and dental mirrors ( Fig. 31-20 ).


Central bars may be amenable to drill excision. Using an intraoperative image from a CT scan, one can place a guide wire into the radiographic center of the bar to be resected. Subsequently, a series of cannulated drills can be passed to remove the bar effectively; I use a series of cannulated reamers used in tunnel drilling for an anterior cruciate ligament (ACL) reconstruction. The defect is then filled with an interposition material, usually autogenous fat. Radioopaque markers are left for the assessment of resumption of growth ( Fig. 31-21 ). Once the bar has been completely resected, the cavity created can be filled with fat or methylmethacrylate (Cranioplast). Polymeric silicone (Silastic) has been used experimentally in humans and animals but is currently unavailable for use. Each of these interposition materials has advantages and advocates. Fat is commonly used because it is readily available and autogenous. Its only drawback is that a separate incision in the gluteal area is often required to harvest a graft of adequate size. Methylmethacrylate is radiolucent and thermally nonconductive. Its solid structure may help support an epiphysis if a large metaphyseal defect has been created. However, it may, be difficult to remove if further reconstructive procedures are required. Some animal studies have investigated biologic interposition materials, although none is currently available for clinical use.

Regardless of which interposition material is selected, the goal is to bridge the physis with the material so that bar formation is prevented. Peterson has advocated contouring the epiphyseal defect or creating drill holes or pods in the epiphysis to anchor the interposition material in the epiphysis so that the interposition material will migrate distally with the epiphysis as growth resumes ( Fig. 31-22 ). Once the bar has been resected and the interposition material has been placed, radiographic markers should be placed on each side of the physis to aid in evaluating the resumption of growth (see Fig. 31-14 ).

Results after bar resection are variable. Almost all authors report poor results with bars involving more than 50% of the physis. It is important to remember that even if growth resumes, premature closure of the physis is common.
Although physeal bar resection clearly has a role in the management of patients with physeal arrest, the surgeon should be cognizant of the relatively modest results and weigh the potential benefits in the context of the actual amount of growth remaining and the cause, location, and extent of the physeal arrest. Close clinical follow-up to maturity is imperative.
Care of the Multiply Injured Child
Blunt trauma is the leading cause of death in children older than 1 year. Although a number of these deaths are caused by such massive injuries that there is no chance of resuscitation, there are deaths that can be prevented with proper trauma care. Although most preventable deaths are the result of pulmonary, intracranial, or intraabdominal lesions, it is important for all physicians, including orthopaedists, who care for victims of acute trauma to be thoroughly familiar with the systematic multidisciplinary approach to the assessment and resuscitation of the polytraumatized child. The principles of assessment and resuscitation have been outlined and well presented in the Advanced Trauma Life Support course provided by the American College of Surgeons. This comprehensive course provides specific training for the management of the pediatric trauma patient.
Anatomic and Physiologic Characteristics Specific to Children
Children possess a number of anatomic and physiologic characteristics that make their injuries and their injury responses different from those of adults. Head and visceral injuries are more common in children, whereas chest and thorax injuries are less frequent. Several factors contribute to the fact that head injuries occur in over 80% of polytraumatized children. First, because a child’s head is relatively large compared with the trunk, the head is usually the point of first contact during high-energy injuries. Second, the cortical bone of the cranial vault is thinner in children. Finally, a child’s brain is less myelinated than an adult’s brain and more easily injured. Fortunately, there are also several characteristics that make recovery from head injury more favorable in children. These include a larger subarachnoid space, greater extracellular space, and open cranial sutures. ||
|| References .
Visceral injuries are also more common in children than in adults, in part because there is less abdominal musculature and less subcutaneous fat. Conversely, the elasticity of the thoracic cage makes fractures of the ribs and sternum uncommon in children. ¶¶ References .
A child’s response to injury is also different from that of an adult’s. It is unusual for children to have preexisting disease, and they usually have large cardiopulmonary reserves. Consequently they can often maintain a normal systolic blood pressure in the presence of significant hypovolemia, although they will develop tachycardia. Children also become hypothermic rapidly because their surface area is large relative to their body mass. This hypothermia can compound the lactic acidosis associated with hypovolemic shock.Management of Airway, Breathing, and Circulation
Evaluation and resuscitation of the polytraumatized child begin with the ABCs ( a irway, b reathing, c irculation) of trauma. Management of the airway should be initiated with the assumption that a cervical spinal lesion exists, and cervical spine precautions should be used until the cervical spine is cleared clinically and radiographically. The relatively large head of a child forces the cervical spine into flexion. Thus appropriate immobilization includes a collar or sand bags as well as a backboard that has a cutout for the head. If these special backboards are unavailable, children may be safely transported by placing a roll under the shoulders to elevate the torso relative to the head ( Fig. 31-23 ). #

# References .
With these cervical spine precautions, an adequate airway must be maintained. The jaw thrust or lift will often open the airway. It is also important to remember that the nostrils must be kept clear in infants. All obvious foreign materials (food, mucus, blood, vomit) must be removed from the mouth and oropharynx. Placement of a nasogastric tube will decompress the stomach and help prevent aspiration. In the unconscious or obtunded child, endotracheal intubation ensures a secure airway.Once an adequate airway has been obtained, breathing and circulation should be assessed. Ventilation should be confirmed by auscultating breath sounds in both lung fields. Absent or decreased breath sounds should alert the surgeon to the possibility of an improperly placed airway or potential pneumothorax. The assessment of blood volume status in children can be deceptive because of their large physiologic reserves. Although children often maintain a normal blood pressure despite significant volume loss, tachycardia develops early in hypovolemic shock. Life-threatening hemorrhage in children is usually the result of a solid visceral injury because children are less likely than adults to sustain massive blood loss from pelvic or extremity trauma. As assessment and management of the airway, breathing, and circulation is being undertaken, attempts should be made to obtain venous access. Once venous access has been established, fluid resuscitation can begin. A child’s circulating blood volume can be estimated as 80 mL/kg. A child’s weight in kilograms can be estimated as weight (kg) = (age [yr] × 2) + 8.
As in adults, fluid resuscitation begins with a crystalloid bolus equal to 25% of the circulating blood volume (20 mL/kg). If tachycardia or other signs of hypovolemia persist after two crystalloid boluses, consideration should be given to transfusion of packed red blood cells. Once fluid resuscitation has begun, the bladder should be decompressed with a Foley catheter. Urine output can then be monitored. Normal urine output in an infant is 1 to 2 mL/kg/hr and 0.5 mL/kg/hr in a child or adolescent.
Primary and Secondary Assessments
The primary survey is completed with a quick history, which should include assessment for medical allergies, current medications, significant past medical history, and details of the accident and management to date. As the primary survey is completed, the secondary survey begins. The secondary survey includes calculation of the Glasgow Coma Scale (GCS) score ( Table 31-3 ) and radiographs of the chest (anteroposterior [AP]), cervical spine (lateral), and pelvis (AP). Additional studies (CT of the head and abdomen, radiography of the extremities and thoracolumbar spine) should be performed as indicated. I obtain AP and lateral radiographs of any extremity that is painful, swollen, ecchymotic, or abraded. Routine blood work should include a complete blood cell count, typing, and crossmatching. It is prudent to draw ample extra blood when venous access is established so that appropriate tests may be added as indicated. The secondary assessment also provides an opportunity to gather information that will allow the computation of an injury score that can be used to classify injury severity and predict morbidity and mortality. A number of scoring systems are available, including the Injury Severity Scale (ISS), Abbreviated Injury Scale, Pediatric Trauma Score, Trauma Score, and Revised Trauma Score. * a
Variable | Score |
---|---|
Opening of the Eyes | |
Spontaneously | 4 |
To speech | 3 |
To pain | 2 |
None | 1 |
Best Verbal Response | |
Oriented | 5 |
Confused | 4 |
Inappropriate words | 3 |
Incomprehensible sounds | 2 |
None | 1 |
Child’s Best Verbal Response | |
Smiles, orients to sound, follows objects, interacts | 5 |
Consolable when crying, interacts inappropriately | 4 |
Inconsistently consolable | 3 |
Moans inconsolably, irritable, restless | 2 |
No response | 1 |
Best Motor Response | |
Spontaneous (obedience to commands) | 6 |
Localization of pain | 5 |
Withdrawal | 4 |
Abnormal flexion to pain | 3 |
Abnormal extension to pain | 2 |
None | 1 |
References .
The Revised Trauma Score is not specific for children; however, it has the advantage of being universally applicable and has been shown to correlate with survival and with the ISS score as well as with the more specific Pediatric Trauma Score ( Table 31-4 ). The ISS score is used primarily for injury classification and outcomes research but also as a measure of quality assurance. It has not been shown to have a direct correlation with mortality. †aRevised Trauma Score | Variables * | ||
---|---|---|---|
Glasgow Coma Scale Score (GCS) | Systolic Blood Pressure (BP; mm Hg) | Respiratory Rate (RR; breaths/min) | |
4 | 13-15 | >89 | 10-29 |
3 | 9-12 | 76-89 | >29 |
2 | 6-8 | 50-75 | 6-9 |
1 | 4-5 | 1-49 | 1-5 |
0 | 3 | 0 | 0 |
* Each of the three variables (GCS, BP, RR) is given a Revised Trauma Score. The Revised Trauma Scores are totaled (range, 0-12). A total score ≤ 11 indicates potentially important trauma.
†a References .
Management of the traumatized child is a multidisciplinary process. As the secondary survey begins, continuous monitoring of airway, breathing, and circulation must continue. Deterioration of vital signs or GCS score may warrant emergency consultation with a neurosurgeon or trauma surgeon. CT of the head is perhaps the single most important study in the management of intracranial trauma. An abdominal CT may often be performed at the same time, with little or no delay. In today’s increasingly specialized environment, the orthopaedist may become involved in the care of a multiply injured child after the initial assessment has begun. One of the advantages of a multidisciplinary approach is that it has a built-in system of checks and balances. Thus the prudent orthopaedist will never assume that the initial assessment has been completed accurately and thoroughly and will begin the assessment with the ABCs and progress to the assessment of any orthopaedic injuries.Perhaps the two greatest mistakes an orthopaedic surgeon can make in managing a traumatically injured child are to assume that a long bone fracture is an isolated injury and that a patient has an unsurvivable injury. At my acute care institution, I routinely consult a trauma surgeon for patients with isolated long bone fractures and a high-energy mechanism of injury (e.g., pedestrians or bicyclists hit by automobiles). In addition, I treat all children aggressively with the expectation that they will recover from even the most severe head injuries. It is important to remember that the secondary survey continues 24 and 48 hours after the injury. Continuous reassessment will help identify the missed injuries noted in 2% to 12% of polytraumatized patients. Unlike in adults, early mobilization is not as important in the management of orthopaedic injuries in a polytraumatized child. ‡a
‡a References .
Nevertheless, orthopaedic injuries should be managed in a fashion that accommodates the needs of all members of the trauma team.A number of recent reports have investigated the development of posttraumatic stress in children after traumatic injury. §a
§a References .
These studies clearly demonstrate that symptoms of posttraumatic stress disorder are common after pediatric orthopaedic trauma. Symptoms have been shown to correlate with more severe injury requiring hospitalization, pulse at presentation, and parental reports of posttraumatic stress. Winston and colleagues have validated a brief screening questionnaire for injured children and their parents.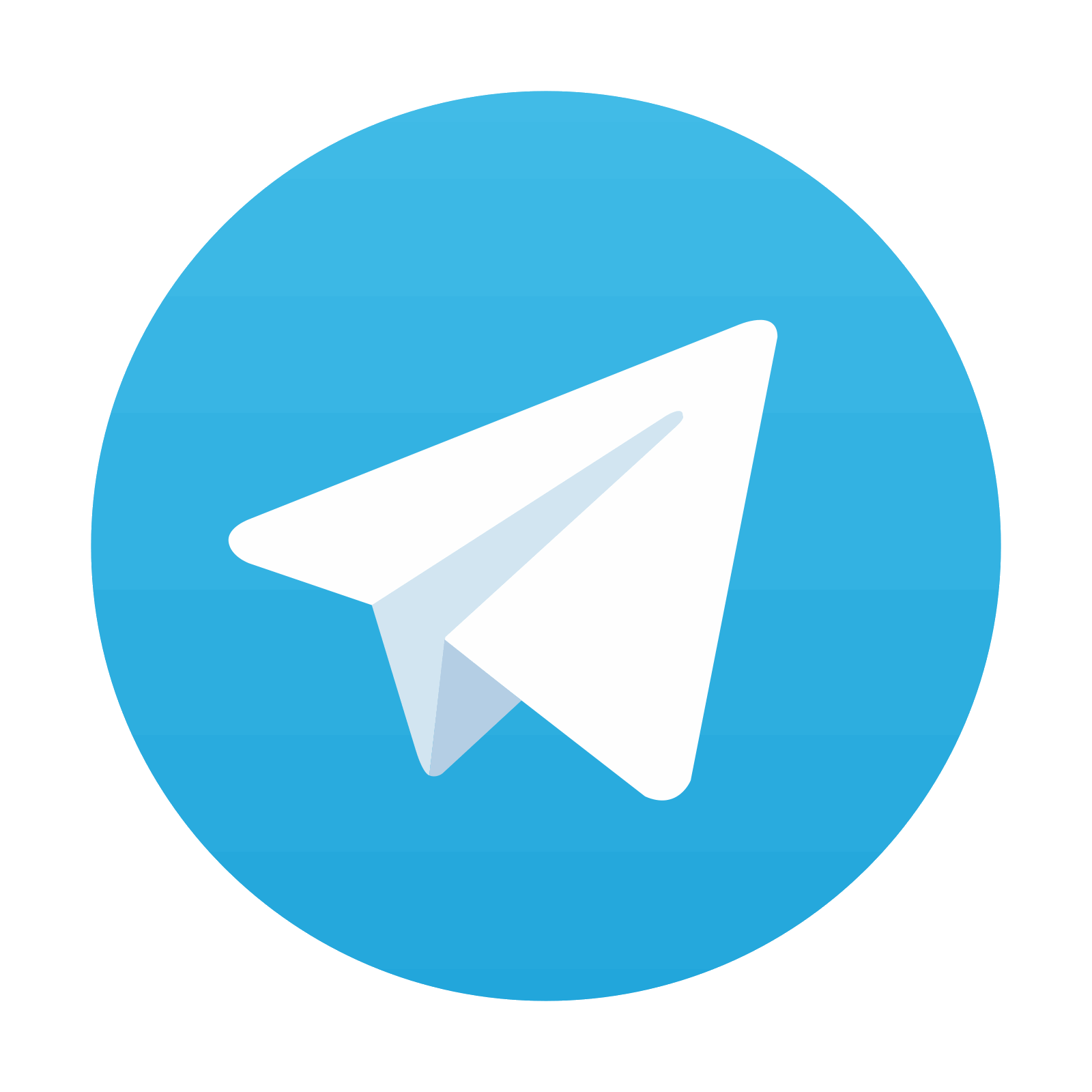
Stay updated, free articles. Join our Telegram channel
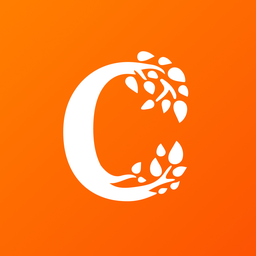
Full access? Get Clinical Tree
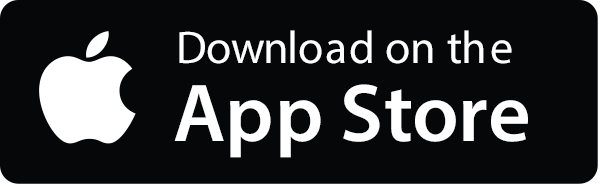
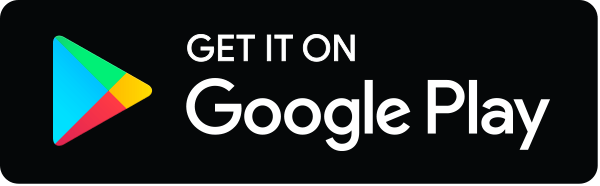