Intervertebral disk degeneration is a common and potentially debilitating disease process affecting millions of Americans and other populations each year. Current treatments address resultant symptoms and not the underlying pathophysiology of disease. This has spawned the development of biologic treatments, such as gene therapy, which attempt to correct the imbalance between catabolism and anabolism within degenerating disk cells. The identification of therapeutic genes and development of successful delivery systems have resulted in significant advances in this novel treatment. Continued investigation of the pathophysiology of disk degeneration, however, and safety mechanisms for the application of gene therapy are required for clinical translation.
Intervertebral disk degeneration (IDD) is a common and potentially debilitating disease process that affects millions in the United States and worldwide. IDD has a variable presentation, from relatively benign to completely disabling, and can be associated with disk herniation, spinal stenosis, radiculopathy, myelopathy, instability, and low back pain. The socioeconomic impact of musculoskeletal disorders of the spine, such as IDD and low back pain, cannot be overstated because these conditions are the leading cause of disability in people 45 years and younger resulting in national economic losses of more than $90 billion annually.
Despite the prevalence of IDD and its enormous socioeconomic impact, operative and nonoperative treatment options are suboptimal and associated with unpredictable outcomes. Current treatment options for IDD and the pathology associated with it include decompression, spinal fusion, diskectomy, electrothermal therapy, and arthroplasty, all addressing the clinical symptoms associated with this disease process (pain and mechanical instability) and not the underlying pathophysiology. With advances in molecular as well as cellular biology researchers have begun to characterize the pathophysiologic pathways associated with disk degeneration and thus provided targets for potential biologic treatments to augment or reverse the course of IDD (see the articles by Leung and colleagues and Bae and colleagues elsewhere in this issue). This article briefly discusses the pathophysiology of disk degeneration, the premise behind gene therapy for the treatment of IDD, strategy for delivery, transfer of therapeutic genes to the disk, and safety concerns associated with its application.
Pathophysiology of intervertebral disk degeneration
The intervertebral disk is the largest avascular structure in the body and is composed of three morphologically distinct regions. The central nucleus pulposus is a gelatinous matrix rich in large aggregating proteoglycans, which imbibe water and may assist in the diffusion of nutrients from the periphery through maintenance of an osmotic gradient. The peripheral annulus fibrosis encases the nucleus pulposus and is a dense fibrotic tissue composed of concentric lamellae rich in type I collagen. The cartilaginous endplates are present at the cranial and caudal aspects of each disk and contain the peripheral vasculature, which nourishes the disk.
In concert these structures absorb and transmit mechanical stress to the vertebrae and surrounding musculoligamentous structures of the spine. Maintenance of the morphologic distinctions between the nucleus pulposus and annulus fibrosis is essential for normal biomechanical function of the disk during loading (see the article by Inoue and Espinoza Orías elsewhere in this issue).
The exact pathophysiology of degenerative disk disease has not yet been completely delineated; however, it is known to be influenced by the interaction between various genetic, biologic, and biomechanical factors. In addition, the morphologic, biochemical, and radiographic changes, which occur with progressive degeneration of the intervertebral disk, have been well characterized. The hallmark of disk degeneration is the progressive loss of proteoglycans, which coincides with decreases in oxygen tension, free radical accumulation, decreased pH, and the increased activity of aberrant proteolytic enzymes. With the loss of proteoglycans, the nucleus pulposus cannot maintain normal physiologic hydrostatic pressure, which results in the dehydration of the disk. In addition, there is a progressive fibrosis of the nucleus pulposus as the ratio between type I and type II collagen increases. As degeneration progresses, the nucleus pulposus and annulus fibrosis lose their morphologic distinction, which ultimately disrupts the finely balanced biomechanics of the disk and spine as a whole ( Fig. 1 ).
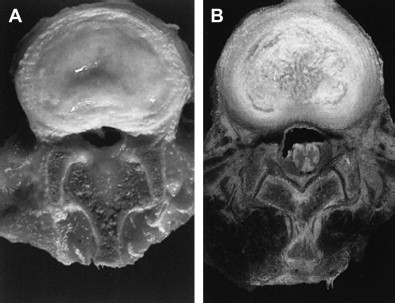
Several studies have indicated that the loss of proteoglycans within the disk is due to an imbalance between anabolism and catabolism within the disk, because proteolytic enzymes (matrix metalloproteinases and A disintegrin and metalloproteinase with thrombospondin) are up-regulated, whereas collagen and proteoglycan synthesis is diminished. Over the past few years, key biologic factors that regulate disk extracellular matrix production, nutrition, cellular proliferation, signaling, and cell death have been identified.
The development of novel biologic treatments, such as gene therapy, have the potential to treat disk degeneration on a molecular level by correcting the biochemical imbalance within degenerating disks and thus potentially changing the natural history of this disease without the morbidity associated with surgery.
Gene therapy
The idea of gene therapy originated as a means to treat heritable genetic disorders by replacing defective genes with functional copies, thus curing the underlying disorder. The current concept of gene therapy has expanded to include the transfer of exogenous genes encoding therapeutic proteins into cells to treat disease. On transduction of this genetic material into the genome of the target cell, the host transcribes the transgene into mRNA, which is then translated by ribosome in the cytoplasm into the desired protein product. These protein products affect not only the metabolism of the host cell but also that of adjacent cells via a paracrine effect. The direct application of therapeutic exogenous proteins is inadequate because their short half-life within the cell precludes any long-term disease-modifying treatment. Gene therapy alters host cell DNA, thereby providing a mechanism for the sustained production of the desired therapeutic product.
The packaging of exogenous genes is a technically demanding and labor-intensive process. First, the enzyme reverse transcriptase is used to construct the complementary DNA (cDNA) of the gene of interest from mRNA. Next, the newly constructed cDNA is cloned in an expression plasmid under the control of an appropriate promoter, which drives the expression of the transgene. Finally, the completed expression plasmid (with the cDNA of the therapeutic gene) is integrated into a vector, which facilitates the entry of the exogenous gene into the host cell.
Gene therapy
The idea of gene therapy originated as a means to treat heritable genetic disorders by replacing defective genes with functional copies, thus curing the underlying disorder. The current concept of gene therapy has expanded to include the transfer of exogenous genes encoding therapeutic proteins into cells to treat disease. On transduction of this genetic material into the genome of the target cell, the host transcribes the transgene into mRNA, which is then translated by ribosome in the cytoplasm into the desired protein product. These protein products affect not only the metabolism of the host cell but also that of adjacent cells via a paracrine effect. The direct application of therapeutic exogenous proteins is inadequate because their short half-life within the cell precludes any long-term disease-modifying treatment. Gene therapy alters host cell DNA, thereby providing a mechanism for the sustained production of the desired therapeutic product.
The packaging of exogenous genes is a technically demanding and labor-intensive process. First, the enzyme reverse transcriptase is used to construct the complementary DNA (cDNA) of the gene of interest from mRNA. Next, the newly constructed cDNA is cloned in an expression plasmid under the control of an appropriate promoter, which drives the expression of the transgene. Finally, the completed expression plasmid (with the cDNA of the therapeutic gene) is integrated into a vector, which facilitates the entry of the exogenous gene into the host cell.
Vectors
The success of gene therapy is dependent not only on sustained expression of the therapeutic gene but also efficient transfer of the genetic material to the host cell. With few exceptions, naked plasmid DNA alone is not an effective means of gene transfer. Therefore, the use of vectors is necessary to facilitate the transfer of genetic information to host cell. There are several types of vectors, most of which fall into one of two distinguishing classes: viral and nonviral.
Nonviral vectors include liposomes, gene guns, DNA-ligand complexes, and microbubble enhanced ultrasound. Liposomes are phospholipid vesicles that deliver the genetic material into the cell by fusing with the host’s cellular membrane. DNA-ligand complexes and gene gun are other nonpathogenic vectors, which are inexpensive and easy to construct. Nishida and colleagues illustrated that ultrasound transfection with microbubbles enhances the efficiency of plasmid DNA uptake into the nucleus pulposus of rats in vivo. The main issue with nonviral vectors is transient transgene expression due to low transfection efficiency into the host genome, thus making these vectors suboptimal for the treatment of chronic diseases in which the sustained production of the desire product is required for disease modification.
Viral vectors use the natural capability of viruses to infect host cells and thus transfer the viral genetic information into the host. Viral vectors are efficient at transducing the desired genetic material to the host cell, even into slowly dividing senescent cellular populations like those of the intervertebral disk. They are also associated with greater risks than nonviral vectors, however, such as cytotoxicity and immune-mediated response. Viral vectors used for gene therapy applications include adenovirus, adeno-associated virus (AAV), herpes simplex virus, lentivirus, retrovirus, and poxvirus. Each viral vector is associated with specific advantages and disadvantages. Therefore, proper selection of vector is critical to successful gene therapy. The most commonly used viral vectors for disk degeneration are adeno and AAV vectors.
Adenoviruses are double-stranded DNA viruses, which have the ability to infect many cell types. There are 47 known human serotypes, of which serotypes 2 and 5 are most commonly used for gene therapy applications. Adenovirus vectors are especially efficient at the transfer of genetic material to host cells, are relatively easy to construct at high titers, and can transduce nondividing quiescent cellular populations. These vectors are easily modifiable for gene therapy applications by the removal of the envelope E1 gene, which is essential for viral gene replication and expression. In addition, the adenovirus genome does not integrate into that of the host cell and remains as an episome within the host cell’s nucleus. Therefore, adenoviruses for gene therapy applications are thought to be associated with low rates of insertional mutagenesis during transduction in comparison with other vectors. The major limitation associated with the use of adenovirus vectors is the short duration of transgene expression in most tissues. This transient expression is attributed to the production of adenoviral antigens by the host cell, with the resulting immune response degrading the adenoviral episome within the nucleus, thus halting therapeutic protein production. In addition, because the adenovirus is not integrated into the host cell’s genome during cellular division, the episome is not replicated. Finally, wild-type adenoviral infections can cause upper respiratory and gastrointestinal illnesses. These issues, although concerning, likely have less clinical relevance due to the asvascular encapsulated nature of the disk. Currently, ways to minimize host adenoviral antigen production and viral protein expression are being investigated.
Unlike adenovirus, AAV is a parvovirus with a single-stranded DNA genome. AAV also has the ability to transfect multiple different cell types, much less efficiently, however, than the adenovirus. There are several distinct differences between adenovirus and AAV. First, the wild-type AAV is not associated with any human disease and thus there are fewer safety concerns with its application. The AAV vector integrates into a specific site on chromosome 19 without damaging the intrinsic genetic material present. Another beneficial feature is that AAV has only two genes (Rep and Cap), which cannot self-replicate and require the presence of a helper virus. Therefore, in the absence of helper virus, there is no expression of intrinsic AAV gene products after transduction, limiting the cell-mediated immune response. The difficulty with using AAV vectors is that they can carry much less foreign (therapeutic) DNA than the adenovirus vector, and the purification of AAV is challenging because the helper virus must be isolated and removed. Despite these shortcomings, AAV has shown real promise as the conduit for successful gene therapy into musculoskeletal tissues. Currently improvements in vector immunogenicity as well as inducible and tissue-specific promoters are being developed. The selection of the appropriate vector is critical for successful gene therapy and depends on disease pathophysiology, therapeutic gene used, and strategy for delivery.
Gene delivery strategy
In addition to the selection of the appropriate gene and vector, another important consideration with gene therapy applications is the delivery strategy used. There are currently two basic strategies for the delivery of exogenous therapeutic genes into target cells. The in vivo strategy involves the direct transfer of the gene-vector complex to the targeted cellular population within the living host. The ex vivo strategy differs significantly because the targeted cells are isolated and removed from the living host. These cells are then cultured with transduction of the therapeutic gene occurring in vitro. The final step includes the reimplantation of the genetically altered cells back into the host ( Fig. 2 ).
Theoretically, the ex vivo method may be a safer approach to gene therapy because the genetically altered cells are observed in vitro; therefore, the cells that show abnormal responses to the transfer of the therapeutic gene can be identified and isolated from the target cell population before reimplantation. There are several disadvantages, however, with the utlization of an ex vivo strategy for clinical gene therapy applications. First, there may be significant morbidity associated with the harvesting as well as the reimplanation of the targeted host cells. Second, often the in vivo environment from which the host cells are removed cannot be replicated in vitro. in vitro, the cells themselves maybe irreversibly altered, not making them suitable to reimplantion. This is of particular concern when considering gene therapy for IDD, because the harsh conditions the cells of the nucleus polposus experience (low oxygen tension, nutrients, and pH) cannot easily be replicated. Additionally in vitro cells are not subjected to biomechanical stimuli, which may be important to cellular signaling and cytokine production. Because of these reasons, most gene therapy applications for disk degeneration use an in vivo strategy for gene delivery.
Modulation of disk cell biology
The foundation for the application of gene therapy to treat disk degeneration is rooted in the identification of exogenous proteins and cytokines, which have shown potential therapeutic benefits, albeit transient, when cultured with disk cells in vitro or directly into the disk in vivo. As discussed previously, several studies suggest that the loss of proteoglycan associated with disk degeneration is due to an imbalance between catabolism and anabolism within the disk. Thus, the goal of gene therapy for the treatment of disk degeneration is to transfer genes, which corrects this imbalance, thereby slowing or reversing the loss of proteoglycans within the disk. Correction of the biochemical imbalances within the disk would likely facilitate the recovery and maintenance of normal disk morphology, thereby improving the biomechanical function of the disk and ultimately altering the natural course of this disease process. In addition, the intervertebral disk is a good target for gene therapy because it is encapsulated and avascular, thus acting as an immune-privileged organ allowing for sustained periods of transgene expression due to the lack of immune response to the therapeutic gene product or the intrinsic proteins produced by the vector.
Thompson and colleagues were the first to demonstrate that in vitro proteoglycan synthesis within the disk could be up-regulated through the application of exogenous human transforming growth factor β1 (TGF-β1) in cultured canine disk tissue. There have been several subsequent studies that have identified other growth factors that have the ability to increase proteoglycan synthesis in intervertebral disk cells. Osada and colleagues demonstrated increased proteoglycan synthesis in cultured bovine disk cells stimulated with exogenous insulin growth factor-1. Takegami and colleagues illustrated a similar increase in proteoglycan synthesis in cultured rabbit nucleus pulposus cells exposed to exogenous osteogenic protein-1, in addition to the recovery of proteoglycan content lost in cells exposed to inflammatory cytokine interleukin (IL)-1. Li and colleagues illustrated that exogenous of bone morphogenetic protein (BMP)-2 not only increased aggrecan expression but also stimulated osteogenic protein-1 expression, further boosting proteoglycan synthesis in cultured rat disk cells. Tim Yoon and colleagues performed an in vitro study on rat intervertebral disk stimulated with BMP-2 and found that this growth factor not only increased cell proliferation and proteoglycan synthesis but also increased the mRNA of type II collagen, aggrecan, and Sox9 genes, which are all chondrocyte-specific genes ( Fig. 3 ). In addition to the anabolic function of these growth factors, Gruber and colleagues demonstrated that the exogenous application of IGF-I and platelet-derived growth factor significantly decreased the number of apoptotic disk cells in culture. Thus, these growth factors may have some utility in treating disk degeneration by maintaining the disk cell population, phenotype, and proteoglycan content.
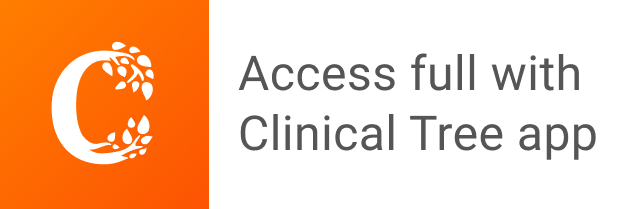