Pearls
- •
The miniaturization and increased efficacy of centrifugal pump systems and low-resistance membrane lungs have resulted in a transition from roller pump devices to centrifugal setups in many centers.
- •
Venovenous cannulation for extracorporeal membrane oxygenation (ECMO) is currently preferred for patients with adequate cardiac function and is used in over 50% of reported children.
- •
Venoarterial access for ECMO remains the most common cannulation technique for patients with cardiac dysfunction and neonates.
- •
In venoarterial ECMO, desaturated venous blood is drained from the body and reinfused into a large artery after being oxygenated in the ECMO circuit. Venovenous ECMO differs from venoarterial ECMO in that blood is both withdrawn and returned into the venous circulation of the patient.
- •
Lack of follow-up hampers efforts to determine long-term neurocognitive and physical functioning following ECMO.
Aided by the discovery of heparin in 1916 and by advances in the technology of membrane lungs, extracorporeal life support has changed dramatically since the early 1950s. Early experiences with the use of cardiopulmonary bypass for operations on the heart were mixed, but as experience and technology have continued to advance, the field of cardiopulmonary bypass has expanded at a rapid rate. As experience with bypass techniques in the operating suite grew, investigation of the use of extracorporeal support of patients with cardiopulmonary failure outside the operating room began. Infants with severe respiratory disease or pulmonary hypertension were among the groups for whom use of a temporary cardiopulmonary bypass system seemed appropriate. This technique of modified cardiopulmonary bypass came to be known as extracorporeal membrane oxygenation (ECMO). Although premature infants had an unacceptably high incidence of intracranial hemorrhage (ICH) as a result of systemic heparinization required to prevent clotting within the extracorporeal circuit, infants of more than 35 weeks’ gestation with respiratory failure were successfully supported with ECMO. An abandoned infant with severe hypoxemia named “Esperanza” (hope) by her caregivers was among the first to be treated with ECMO by Robert Bartlett in 1976. Today, Esperanza is a grown woman with children of her own.
Efforts to organize and collate data on patients treated with ECMO resulted in the formation of the Extracorporeal Life Support Organization (ELSO). In 2019, the ELSO celebrated its 30th anniversary. This largely volunteer network of physicians, surgeons, nurses, respiratory therapists, and all those with interest in extracorporeal life support comprises more than 300 centers and contains data on more than 130,000 patients treated with extracorporeal life support throughout the world. The recent rise in new ECMO centers and support of older children and adults is exciting; unfortunately, many patients who receive ECMO are not reported to the ELSO, however. This hampers efforts to obtain a fully accurate description of ECMO use and outcomes throughout the world. As more patients have been treated and techniques have been refined, ECMO procedures and management of patients have evolved to the point that ECMO can now be offered to patient groups previously excluded from consideration. The decision regarding when a patient should be treated with ECMO remains empirical and is often based on clinical judgment and case discussion with involved clinicians and family. Similarly, although there is little complete standardization of ECMO circuit design, cannulation technique, and patient management, the general principles are fairly consistent. Guidelines for ECMO center training, equipment selection, and patient selection and management have recently been developed by expert consensus and are posted on the ELSO website ( www.ELSO.org ). Although the guidelines are general, the expectation is that they will enable more standardization of practice in the future. The information in this chapter represents general practice, our experience, and a review of the literature. For more detailed information regarding extracorporeal life support, the reader is directed to the excellent text regarding this subject published by the ELSO organization.
A trip around the extracorporeal membrane oxygenation circuit
Adequate ECMO support begins with selection of the appropriate cannulas and circuit to provide required flow for circulatory support and oxygen delivery (D o 2 ). A guideline for selection of cannula size and circuit components based on patient weight is shown in Table 56.1 . Use of ultrasound to help size vessels and select cannulas that can fit is useful. Multiplying the cross-sectional width of the vessel in millimeters by a factor of 3 will estimate the appropriate French size (Fr). Ultrasound can also identify whether the vessel is patent for cannulation. Cannulation is usually performed at the bedside or in the operating theater, with the patient receiving a combination of local anesthetics and intravenous analgesics, sedatives, and neuromuscular blocking drugs. An initial bolus of heparin (usually 50–100 U/kg) is given just prior to vessel cannulation to prevent clotting of the inserted cannulas before initiation of ECMO flow. ECMO flow is initiated and is increased in increments over the first few minutes to prevent sudden changes in hemodynamics that may adversely affect cerebral blood flow or cardiac function. In infants, a rate of 100 to 150 mL/kg per minute usually provides adequate perfusion and oxygenation. Use of high-flow ECMO (up to twice that estimated) also is recommended in patients with single-ventricle physiology and a systemic to pulmonary artery shunt to provide adequate circulation for both systemic and pulmonary organs. Pediatric patients usually require about 90 mL/kg per min of ECMO flow to maintain adequate D o 2 ; adult patients require rates of 50 to 70 mL/kg per minute. Estimates of flow needs also can be predicted by using cardiac index data based on body surface area (BSA). One caution in estimating ECMO flow is that patients with sepsis or multiple organ dysfunctions may require flows that are much higher than predicted. These factors must be considered when selecting cannula size, as larger cannulas than that predicted by BSA may be required.
Patient Weight | Neck, Reinfusion | Groin | Internal Jugular Cannulation | ||
---|---|---|---|---|---|
Reinfusion | Drainage | Drainage | Reinfusion | ||
2–4 kg | 13 Fr WR-Origen DL | 13 Fr Wr-Origen DL | 13 Fr Avalon (if available) | ||
4–7 kg | 13–16 WR Origen DL | 13–16 WR Origen DL | 13 Fr Avalon (if available) | ||
7–10 kg | 16–19 WR Origen DL | 16–19 WR Origen DL | 16–18 Fr Avalon | ||
10–12 kg | 19 Fr WR Origen | 17–19 Fr Biomedicus | 15 Fr Venous Biomedicus | 12 Arterial Edwards or Biomedicus | 19 Fr Avalon |
12–14 | 19 Fr Origen WR DL | 19 Fr Origen WR DL | 15 Fr Venous Biomedicus | 14 Arterial Edwards | 19 Fr Avalon |
15–16 kg | 15 Arterial Biomedicus | 17 Venous Biomedicus | 14 Arterial Edwards | 19–21 Avalon | |
17–20 kg | 15 Arterial Biomedicus | 19 Venous Biomedicus | 15 Arterial Biomedicus | 19–21 Avalon | |
21–25 kg | 15 Arterial Biomedicus | 19 Venous Biomedicus | 15 Arterial Biomedicus | 21–23 Avalon or 24 Crescent | |
26–30 kg | 15 Fr Arterial Biomedicus | 15 Fr Venous Biomedicus | 21 Fr Venous Biomedicus | 15 Fr Arterial Biomedicus | 23 Fr Avalon/24 Fr Crescent/29 Fr Protek Duo |
31–35 kg | 15 Fr Arterial Biomedicus | 15 Fr Venous Biomedicus | 21 Fr Venous Biomedicus | 15 Fr Arterial Biomedicus | 23 Fr Avalon/24 Fr Crescent/29 Fr Protek Duo |
36–40 kg | 17 Fr Arterial Biomedicus | 17 Fr Venous Biomedicus | 23 Fr Venous Biomedicus | 17 Fr Arterial Biomedicus | 27 Fr Avalon/24 Fr Crescent/29 Fr Protek Duo |
41–45 kg | 19 Fr Arterial Biomedicus | 17 Fr Venous Biomedicus | 23 Fr Venous Biomedicus | 17 Fr Arterial Biomedicus | 27 Fr Avalon/24 Fr Crescent/29 Fr Protek Duo |
46–50 kg | 19 Fr Arterial Biomedicus | 19 Fr Venous Biomedicus | 23 Fr Venous Biomedicus | 17 Fr Arterial Biomedicus | 27 Fr Avalon/24 Fr Crescent/29 Fr Protek Duo |
51–60 kg | 19 Fr Arterial Biomedicus | 19 Fr Venous Biomedicus | 23 Fr Venous Biomedicus | 19 Fr Arterial Biomedicus | 27 Fr Avalon/28Fr Crescent/31 Fr Protek Duo |
61–65 kg | 19 Fr Arterial Biomedicus | 19 Fr Venous Biomedicus | 23 Fr Venous Biomedicus | 19 Fr Arterial Biomedicus | 31 Fr Avalon/28Fr Crescent/31 Fr Protek Duo |
66–70 kg | 19–20 Fr Arterial Biomedicus | 19 Fr Venous Biomedicus | 23 Fr Venous Biomedicus | 21 Fr Arterial Biomedicus | 31 Fr Avalon/30Fr Crescent/31 Fr Protek Duo |
>70 kg | 19–20 Fr Arterial Biomedicus | 19 Fr Venous Biomedicus | 23 Fr Venous Biomedicus | 21 Fr Arterial Biomedicus | 31 Fr Avalon/30Fr Crescent/31 Fr Protek Duo |
Cannulation techniques
By convention, the drainage site is indicated by the abbreviation of the cannulation mode: venoarterial (VA), venovenous (VV), arteriovenous (AV), or venoarteriovenous (VAV). The primary modality to verify proper cannula position has traditionally been chest radiography. However, echocardiography is more accurate and should currently be considered the gold standard for evaluation of cannula placement. Differences between VA and VV support are shown in Table 56.2 .
Venoarterial | Venovenous |
---|---|
Nonpulsatile flow | Pulsatile flow (normal flow through body) |
Increased afterload | No change afterload |
Decreased preload | No change preload |
Venous blood to lungs | Oxygenated blood to lungs |
Artery ligation/stenosis/distal ischemia | No arterial access |
Microemboli to body | Microemboli to lungs |
Direct cardiac support | Indirect cardiac support Requires adequate cardiac function |
Patient Sa o 2 95% to 100% | Patient Sa o 2 75% to ≥85% |
Venoarterial extracorporeal membrane oxygenation ( fig. 56.1 a)
Venous cannulation
The right atrium provides the largest repository of venous blood for access and is the usual site accessed for venous drainage. The internal jugular (IJ) vein is a large vessel with a short, straight course to the right atrium and is thus preferred during cervical cannulation. Proper placement has cannula drainage holes within the right atrium; often, measuring from the insertion site to the level of the xiphoid results in good position. On chest radiograph, the cannula may be visualized close to the diaphragm. To augment cerebral venous drainage in patients who undergo cannulation via the right IJ vein, some centers also place a smaller cannula retrograde in the vessel to the level of the jugular venous bulb at the base of the skull. This catheter is then connected by a Y-adaptor into the venous drainage line. Some centers have found that venous drainage can be increased up to one-third in infants, and increased flow for older children has also been reported. ,




In older children and adults, the femoral veins can be used for cannulation. The cannula can be advanced up the inferior vena cava (IVC) to the level of the right atrium, or even further to the level of the superior vena cava (SVC)/right atrial junction. Although femoral vein cannulation diverts less venous return to the pump because the length of the cannula and the smaller Fr increase resistance to blood flow more so than IJ access, the amount of blood drained is often adequate to meet the needs of the patient. Femoral cannulation is generally restricted by vessel size to patients who weigh at least 15 kg. Compartment syndrome from venous stasis in femoral venous cannulation has been described; thus, careful monitoring of the extremity is mandatory.
In patients who undergo cannulation via the mediastinum, the venous cannula is often placed directly into the right atrial appendage. Other vessels, such as the subclavian or axillary vessels, have been associated with limb perfusion abnormalities or difficulties with adequate blood flow in the past. New cannulas with better flow dynamics or use of side grafts onto vessels that do not occlude distal blood flow may allow for the use of vessels that have been previously avoided.
Arterial access
In infants, cannulation of the right carotid artery to the arch of the aorta is the preferred route. Depth of cannula insertion can be estimated by measuring the distance from the insertion site to the level of the sternal notch. Care must be taken not to position the end of the cannula close to the aortic valve, because this position may result in aortic insufficiency induced by the high-velocity blood flow returning from the ECMO circuit and being directed toward the aortic valve leaflets. Alternatively, if the arterial cannula is advanced too far down the aortic arch, it can occlude blood flow to the left carotid artery and the brain.
In mediastinal cannulation, the arterial return cannula is usually placed into the aortic arch under direct vision, or cannulas that were used during cardiopulmonary bypass if the patient is transitioning directly from bypass to ECMO can also be used.
Use of the femoral artery for access during VA ECMO is common, especially in adults or in large patients requiring emergent access such as during cardiac arrest. Femoral arterial cannulation also can be associated with impaired flow to the distal limb and resultant ischemia. , A small “feeder” cannula can be directed distally down the leg artery and then connected by a Y adapter into the arterial cannula to improve perfusion. Additionally, placement of a 14-gauge catheter into the posterior tibial artery that is then connected by a Y adapter into the arterial side of the ECMO circuit has been reported. Ischemia and the need for amputation with femoral artery cannulation occurs even with such precautions; therefore, meticulous attention to limb perfusion is required to prevent this complication. Doppler assessment of pulses and use of near-infrared spectroscopy on each lower extremity are sometimes simple means to follow if flow is adequate past the cannulation site. Establishing a graft onto the femoral artery through which the cannula is inserted, ultrasound-based sizing of the femoral vessels, and use of a cannula that will not completely occlude the femoral artery have also been suggested to limit risk of ischemia. In larger patients, side-graft techniques have also been used successfully to allow ECMO support via subclavian or axillary vessels. These techniques are especially useful in patients being bridged to lung or heart transplant, as they can be “tunneled” under the skin and allow better patient mobility and reduced infection risk. Hyperemia of the limb distal to the returning ECMO flow may occur and require partial occlusion of the distal artery.
Left heart decompression
As VA ECMO increases afterload to the left heart, patients with severe left ventricular function may develop complete failure on initiation of ECMO. As ECMO is performed in a “partial” bypass manner and blood from Thebesian and bronchial vessels returns to the left heart, adequate left heart function is required to expel collected blood. Failure to open the aortic valve to eject blood creates left heart distention, left atrial hypertension, pulmonary venous hypertension, and acute pulmonary edema—or frank hemorrhage—can follow. Patients with mediastinal cannulation may have a left atrial venting catheter inserted to allow decompression of the left heart. This catheter can be connected by a Y adapter into the venous drainage of the extracorporeal life support (ECLS) circuit to provide adequate left heart decompression.
Patients with intact sternums who require left atrial decompression often are taken to the cardiac catheterization suite for a blade atrial septostomy, which allows the left heart to decompress into the right atrium and the blood to be drained into the venous ECMO cannula. Experience with use of an Impella device to provide left heart drainage or use of an intraaortic balloon pump (in larger patients) has also been successful.
Venovenous extracorporeal membrane oxygenation
VV ECMO differs from VA ECMO in that blood is both withdrawn and reinfused into the patient’s venous circulation (see Fig. 56.1 B); thus, adequate cardiac function must exist. Cannulation can be introduced via either the cervical or femoral vessels, although new cannulas with improved flow dynamics allow for the use of other vessels as well. Currently, several types of multiple-lumen single cannulas exist. One such cannula, manufactured by Origen, is potentially available in sizes from 13 to 32 Fr and has been used often in infants. This cannula is placed into the right IJ vein and requires only one surgical site. The drainage and infusion lumens in this cannula are separated by a distance of a few centimeters. Careful placement and orientation of the cannula can reduce recirculation of reinfused blood from the ECMO circuit, although some amount of recirculation (which will be discussed later in further detail) always occurs. Other cannulas, especially popular for use with adults and larger children, are manufactured by Maquet (Avalon) and Medtronic (Crescent), and are up to 32 Fr. These cannulas have two drainage lumens, one of which is positioned in the IVC and one in the SVC. A reinfusion port that sits between the two lumens is directed at the tricuspid valve when placed properly, thus limiting recirculation of oxygenated blood. Placement of this cannula requires meticulous assessment with echocardiography or fluoroscopy for safe and optimal performance. Although experience with the Avalon cannula has been good, especially among adolescents and adults, late cardiac perforation or difficulty in maintaining proper placement has been reported in patients receiving the 13 Fr cannula, which has been reconfigured. Effectiveness is not yet well documented. Limited availability of small cannulas for VV ECMO in neonates and infants is still resulting in many patients receiving VA support. The Crescent (24–32 Fr) has limited patient experience at this writing, but initial results are favorable. The Crescent has a slightly larger return orifice, reinforced suture collars, and radiopaque markers to identify SVC, IVC, and return port sites. Time will tell whether the advantages of these cannulas outweigh their increased cost. Obstruction to venous drainage and deep vein thrombosis in the upper extremities are complications that may occur.
VV ECMO also can be provided via two (or more) separate access sites; this is the most popular mode in many centers. Patients with VV cannulation may have venous blood drained from the right atrium via the IJ vein or from the IVC via the femoral vein. Although more venous drainage usually can be obtained from a cervical cannula (which is usually shorter and larger than a cannula that can be placed into the femoral vein), the femoral site often may prove adequate, and draining from the femoral vessel and reinfusing into the IJ results in less recirculation. Older children and adults also may undergo bilateral femoral cannulations, with one cannula placed into the high IVC and the other ending in the low IVC or iliac vessels.
Venoarteriovenous extracorporeal membrane oxygenation
The venoarteriovenous (VAV, sometimes also referred to as venovenoarterial) mode is a hybrid mode that combines VV support for pulmonary failure and partial VA support, with the advantage of percutaneous cannulation. The typical circuit consists of a drainage cannula in the right femoral vein and two return cannulas connected via a Y connection, one in the right IJ vein and the second in a femoral artery. Alternatively, a dual-lumen IJ venous cannula and carotid or subclavian arterial cannula can be used, which simplifies access. Blood flow between the two return limbs is controlled to achieve approximately 30% flow into the arterial system by restricting the flow to the venous cannula (usually with a simple screw clamp). VAV support is ideally suited for larger pediatric patients who require partial cardiac support in addition to pulmonary support.
Arteriovenous
The concept of pumpless AV support of gas exchange was described over 40 years ago but was not feasible until the development of low-resistance, high-efficiency membrane lungs. Usually placed in the femoral artery and vein, the patient’s systemic AV pressure gradient drives flow through a membrane lung without the need for a pump. Because arterial blood is usually well oxygenated, oxygen transfer is limited, but carbon dioxide (CO 2 ) is readily removed. The applications of AV CO 2 removal (AVCO 2 R) are identical to those of extracorporeal carbon dioxide removal (ECCO 2 R) without the need for an extracorporeal pump, that is, reduction in mechanical ventilatory support and control of hypercapnia. A blood flow of 15% to 20% of cardiac output is required, and, in adults, a cardiac index of 3L/min per m 2 and mean arterial blood pressure greater than 70 mm Hg are considered necessary to avoid the need for a pump in this method of support. Another form of pumpless ECMO support is used in patients with severe pulmonary hypertension. This form of paracorporeal lung assist uses the right ventricle to push blood flow via a cannula placed in the pulmonary artery through the low-resistance membrane lung where CO 2 is removed and oxygen added. The oxygenated return flows via a cannula placed in the left atrium and native heart ejection provides systemic blood flow. Patients can be supported until their acute pulmonary hypertensive crisis is over or even to the point of heart/lung transplantation.
Percutaneous cannulation
Although the historical approach to vessel access has been via an open procedure with placement of cannulas under direct vision, kits for percutaneous placement now exist in many sizes. Percutaneous cannulation avoids the need for an open surgical site, thus decreasing surgical-site bleeding and risk of infection.
Ultrasonography is also helpful in guiding needle entry during vessel puncture, enhancing the chance of successful cannulation. Use of fluoroscopy during percutaneous catheter insertion can help to identify aberrant guidewire placement and reduce the chance of vascular injury.
Percutaneous kits use a modified Seldinger technique with obturators increasing in size to dilate the vessel. Once the appropriate size is reached, the cannula is passed into the vessel over the largest obturator.
Percutaneous cannulation carries with it the inherent risk of potentially tearing a large vessel during cannulation. Although percutaneous cannulation in some centers is performed by nonsurgical personnel, surgical backup to perform an immediate cutdown for control of bleeding from a disrupted vessel may be needed. Despite the obvious fear of vessel disruption, this complication occurs infrequently. In one series of 100 patients who were cannulated by nonsurgical personnel, only two vascular complications occurred. Both were associated with mortality. A more recent experience with 32 ECMO runs in neonates and children (3.0–17 kg, 13–27 Fr double-lumen cannulas) with percutaneous cannulation by intensivists also noted successful placement with no major adverse events.
Extracorporeal membrane oxygenation circuit
The majority of ECMO circuits are incorporated with the ECMO pump and membrane lung, which makes setup and priming easier, although some require assembly. Circuit configuration can be tailored for centers specifically as well. Most manufacturers provide coating of their circuits to help prevent platelet activation and aggregation, although this has not totally prevented use of anticoagulation and the success of the surface coating is not well proven. Over time, the complexity of circuits and access ports has been reduced to limit accidental exposure to air embolus from open stopcocks, especially as centrifugal systems have come into practice. The number of connectors within the circuit has also been linked to fibrin deposition and hemolysis and may increase the risk of infection.
Venous reservoir and venous saturation monitor
In cardiopulmonary bypass, use of an open venous reservoir allows venous filling pressures to be controlled and prevents excessive negative pressure in the bypass circuit to develop as blood is withdrawn from the heart. ECMO operates in a closed system; thus, generation of high negative pressure as blood is withdrawn can be transmitted to the venous cannulation vessel. This can induce endothelial damage, destruction of red blood cells with resultant hemolysis, or cause a decrease or cessation in venous return to the ECMO circuit. To prevent these events, most centers use either a servoregulated pressure monitoring system that decreases or stops pump flow if excessive negative pressure is measured or employ a smaller version of a venous reservoir commonly referred to as a bladder . In roller head devices, the bladder sits at the lowest point of the ECMO circuit and allows blood to collect and be drawn from it to the pump head. It also acts as an air trap and normally has access ports to allow aspiration of any air collected in the bladder chamber. The bladder can be used as a servoregulating device that helps to match forward flow to venous return. Servoregulation by monitoring of direct negative and positive pressure within the ECMO circuit has eliminated the use of the bladder in many centers, although those that use roller pump systems most often still incorporate some sort of bladder within the circuit.
Another important feature of the ECMO circuit is the venous saturation monitor, which is placed along the venous drainage line. Most new pump systems incorporate this into their monitoring apparatus. Monitoring venous saturation over time gives information regarding the balance of D o 2 and extraction. Use caution when interpreting venous saturation in the presence of left atrial drains or left-to-right intracardiac shunts in patients with adequate pulmonary function and gas exchange, as oxygenated blood returning to the left atrium will be directed into the venous drainage line and elevate observed venous saturation. To obtain a more accurate assessment of the patient’s venous saturation, monitoring should occur at a site proximal to entry of oxygenated blood into the circuit.
Priming
Although ECMO can be initiated with a crystalloid prime in infants or in circumstances in which ECMO is not emergent, blood priming may be optimal. Because blood has been citrated and stored, it may be acidotic, depleted of calcium, and have a high potassium level. Calcium (usually as calcium chloride), bicarbonate or tromethamine, and heparin are added during the blood priming procedure. Electrolytes should be measured in the priming blood before bypass is initiated because disturbances of cardiac rhythm, or frank cardiac arrest can occur upon initiation of ECMO. Hyperkalemia exists almost universally in the blood-primed circuit despite buffering by calcium and bicarbonate. The potassium level rarely causes systemic effects once the ECMO prime is diluted with the patient’s intrinsic blood volume. Use of the freshest blood available also may lessen the degree of hyperkalemia in the primed circuit. Rarely, hyperkalemia may be of such concern that blood must be washed prior to ECMO use or filtered via a hemofiltration device in the ECMO circuit to reduce the potassium level. Newer centrifugal/hollow fiber systems have a priming volume much lower (<400 mL) than with older roller pump/silicone lung configurations and may also decrease the risk of hyperkalemia. It should be noted, however, that sudden changes in cardiac rhythm or the “myocardial stun” phenomenon whereby the heart seems to stop contracting on initiation of ECMO are often related to hypocalcemia (or hyperkalemia). Medical management of these conditions must be implemented immediately.
Types of pumps and oxygenators
Roller head pumps
The majority of ECMO prior to 2012 was performed using a roller head semiocclusive pump. Venous blood is siphoned via gravity to the roller heads, which are enclosed in a box (the pump housing). Compression of the tubing inside the pump housing (called the raceway ) by the rollers advances blood forward at high pressure to the membrane lung from which it is returned to the patient. The gravity siphon, the need to periodically reposition tubing within the raceway to avoid rupture from excessive spallation, and the need for pressure monitoring with multiple access points within the circuit combine to require a large priming volume. This increases the exposure to blood products and the associated inflammatory response from contact with the artificial surface of the circuit. Hemolysis from red cell destruction within the raceway or other areas of turbulent flow in the circuit may also occur. Roller pumps generate high pressure in the circuit distal to the raceway/roller heads. Thus, acute interruption to forward flow as may occur with kinking of the arterial cannula or elevated resistance to blood flow on the high-pressure side of the circuit can result in immediate and potentially lethal circuit rupture. Monitoring of the high-pressure side of the ECMO circuit is universal, with critical high limits for arterial line pressure determined based on tubing size and pump flow. Pressures below 300 to 350 mm Hg are desired; safety mechanisms will stop the ECMO pump if line pressure limits are exceeded. As air embolus into the patient can be lethal (especially with VA support), servoregulation to decrease or stop the pump if air bubbles are detected are often incorporated into the circuit. Newer pumps may provide pulsatile flow. The nonpulsatile nature of VA ECMO flow has been implicated in the renal dysfunction that is sometimes noted in patients treated with this modality. The pulsatile flow of the newer pumps has yet to be effectively linked to native heart ejection, although this remains a goal for the future. ,
While roller pump systems were the primary reported method of ECMO support until 2012, advances in centrifugal technology have resulted in many centers, especially outside the United States, eliminating roller pump ECMO. At this writing, 600 to 700 patients still receive roller pump ECMO yearly, the majority being neonates.
Centrifugal pumps
Centrifugal devices contain a magnetically controlled spinning head. Blood enters via the ECMO circuit at the inner apex of the pump head and is propelled tangentially to the outer wall where it is ejected into the circuit and advances to the membrane lung and back to the body. The longer blood sits in the centrifugal head, and the faster the head spins, the more red blood cells are exposed to shear stress, and hemolysis may be created. In the past, centrifugal heads were large and heat was generated by the rotating head seated on a bearing. Because blood remained in the head longer at the low flow rates that infants require, hemolysis was severe. Although newer models have small heads with small priming volumes (e.g., 13 mL) and levitation may create less heat, some data have shown that hemolysis is still high at infant flow rates while other research has found patient size and flow rates not to be important factors. It is also postulated that, as clots develop in the membrane lung over time and increase outflow resistance, hemolysis worsens in the rotor head. Centrifugal pumps may also create microemboli that can reach the patient if they are not trapped in the membrane lung. Air bubble detectors are a component of most centrifugal systems. Centrifugal pumps have been linked to renal failure and other adverse events in infants in some recent studies, although concerns that the learning curve to adjust to centrifugal support from roller head systems may be related to observed outcomes and not necessarily the pump itself. Centrifugal pumps are very sensitive to preload and afterload; understanding the physiology of their operation is mandatory to providing optimal ECMO support. , , Despite some concerns, centrifugal pumps have many features that make them potentially safer and easier to operate than roller pump systems. They do not require gravity drainage; thus, they can be placed at any level as long as it is lower than the patient’s heart, and therefore circuit lengths can be shorter. As centrifugal pumps advance blood only if the downstream pressure is less than that generated in the circuit, the absence of risk of tubing rupture if postpump sites are occluded is another advantage. They are easier to transport, have a smaller footprint, and many have built-in pressure and flow monitoring systems that can help servoregulate flow. These factors make centrifugal technology theoretically safer than roller devices and have fostered transition to a “single caregiver” model in some centers, which reduces or eliminates a specific ECMO specialist to monitor the system on a 24/7 bedside basis and has the bedside nurse provide both patient care and ECMO circuit oversight. ECMO experts are available for troubleshooting or intermittent rounding purposes. Since 2012, the use of centrifugal pumps has exceeded that of roller systems, and they have replaced roller systems in most countries outside the United States.
Negative inlet pressure
Both roller and centrifugal pumps can create high negative pressure on the venous inflow side (also referred to as the inlet ). Loss of venous return with continuous rotation of either roller head or centrifugal pumps results in generation of high negative pressure in the ECMO tubing that can lead to hemolysis and cavitation as air is drawn out of solution. As mentioned, blood flow to the centrifugal head is augmented by the “suction” effect of the spinning pump head and thus is not as dependent on gravity drainage as are roller head pumps. The active suction effect of centrifugal heads can create levels of negative pressure as high as −200 to −700 mm Hg within the venous inlet tubing. Monitoring of venous pressure with alarm limits to signal when excessive negative pressure is occurring is common in most centers. Setting an appropriate venous line or inlet pressure is an important part of circuit management but seems frequently misunderstood. Optimal servoregulation alarms or interventions require several factors that should be taken into consideration. The most important fact is that the total resistance on the venous side of the circuit is the sum of all resistances encountered. The major contributor to this negative pressure is the pressure drop across the venous cannula. Most manufacturers provide pressure drop curves for each of their cannulas across a range of flow rates. While these curves are created using water instead of blood—and thus do not represent the effect of viscosity of blood—they provide a suitable surrogate for the resistance induced by the cannula. Selecting a cannula that will provide the expected blood flow at a pressure drop of less than 100 mm Hg is recommended. Pressure drops greater than 100 mm Hg will result in an increased risk of hemolysis. Another factor to consider is that animal research has shown that exposure of the right atrium to pressures more negative than 20 mm Hg can result in damage to the intima. Taking these two points into consideration, if the venous cannula chosen to provide the expected blood flow rate has a pressure drop of 40 mm Hg from inlet to outlet, the venous servo limit should be set at −60 mm Hg to protect the right atrium from damage and provide adequate blood flow. Thus, the venous pressure limit to be set is not a constant number but will vary from patient to patient based on cannula size and desired flow rates. Clinicians also vary as to where best the venous pressure should be measured—at the junction of the catheter to the circuit tubing, which will reflect negative pressure closest to the heart, while others use the prepump-head site, which reflects the most negative pressure within the total circuit.
Servoregulation of both inlet and outlet pressures are set, and the pump (or specialist) adjusts the revolutions per minute of the pump head or the desired flow to maintain set goals.
Membrane lung
Until recently, the predominant membrane lung was a silicone membrane envelope (with a plastic spacer screen inside) wound in a spiral fashion around a polycarbonate spool. , Gas flows within the interior of the envelope, and blood flows between the turns in the membrane envelope. Gas exchange occurs due to partial pressure differences in the gas and blood. While this device is commonly referred to as the oxygenator , CO 2 removal is also provided—thus, the correct term should be membrane lung . Blood flow to the membrane lung is controlled by the pump setting. While the silicone lung was extremely efficient in gas exchange, it had high resistance to blood flow; newer versions of the membrane lung have replaced it in ECMO support.
Hollow-fiber membrane lung
Almost overnight, new hollow-fiber membranes replaced the silicone lung during ECMO use. , Early versions of these oxygenators were plagued by difficulties with plasma leakage that resulted in very short life spans for the device and limited their use. The development of polymethylpentene membranes that are extremely resistant to plasma leakage and have excellent gas exchange characteristics has made them the preferred device. These devices have a low resistance to blood flow and a lower priming volume than the traditional silicone membrane lung, which makes them faster and easier to prime. Newer versions maintain excellent gas exchange, have little hemolysis, and have durability for days to weeks without failure. In the United States, the Quadrox device (Maquet, Inc.) and the Medos lungs (Fresenius) are currently available. Other countries use similar devices. These new, low-resistance, hollow-fiber membrane lungs allow easy propulsion of blood from the centrifugal device and are a major reason that centrifugal pumps/hollow-fiber membrane lung devices have become so popular for patients of all ages. ,
A recent evaluation of pumps and oxygenators revealed that, in over 500 patients from 2012 to 2014, centrifugal pumps were used in 67% of patients and polymethylpentene membrane lungs in nearly 100%.
Patient populations treated with extracorporeal life support
Neonatal cardiopulmonary failure
The majority of ECMO patients reported to the ELSO registry were neonates with respiratory failure ( Table 56.3 ). , The advent of inhaled nitric oxide, surfactant, high-frequency ventilation, and improved pre- and postnatal care has decreased the frequency of ECMO use in these small patients. Nonetheless, 800 infants, on average, receive ECMO each year. While neonatal respiratory distress syndrome has virtually disappeared, patients with meconium aspiration syndrome, persistent pulmonary artery hypertension of the newborn and sepsis still require ECMO support. These infants experience a combination of pulmonary parenchymal and vascular dysfunction that leads to impaired gas exchange. The diagnosis and outcome of ECMO applied in neonatal patients are shown in Table 56.4 .
NUMBER (% SURVIVAL) | ||||
---|---|---|---|---|
Total Runs | Venoarterial | Venovenous | Survived to DC | |
Neonatal | ||||
Respiratory | 31,591 | 2917 (63) | 1124 (77) | 23,119 (73) |
Cardiac | 8252 | 2917 (63) | 1124 (77) | 3529 (43) |
ECPR | 1864 | 756 (41) | 3 (33) | 775 (42) |
Pediatric | ||||
Respiratory | 9487 | 1196 (53) | 1863 (69) | 5573 (58) |
Cardiac | 11,377 | 3824 (58) | 67 (56) | 5980 (52) |
ECPR | 4361 | 1945 (43) | 36 (52) | 1858 (42) |
Adult | ||||
Respiratory | 19482 | 1177 (50) | 12,395 (61) | 11,565 (59) |
Cardiac | 19627 | 14054 (43) | 509 (43) | 8381 (42) |
ECPR | 6190 | 4572 (29) | 121 (41) | 1827 (29) |
TOTAL | 112,231 | —- | —- | 62,607 (55) |
Diagnosis | Total Runs | Average ECMO Duration Hours (Longest) | % Survived |
---|---|---|---|
Neonatal | |||
CDH | 1253 | 311 (1733) | 50 |
MAS | 718 | 146 (2286) | 92 |
PPHN | 679 | 168 (1154) | 73 |
RDS | 28 | 127 (562) | 82 |
Sepsis | 118 | 182 (1155) | 50 |
Pneumonia | 32 | 364 (982) | 53 |
Air leak syndrome | 9 | 130 (282) | 88 |
Other | 1065 | 182 (1558) | 69 |
Pediatric | |||
Viral pneumonia | 330 | 308 (1680) | 71 |
Bacterial pneumonia | 198 | 327 (4286) | 70 |
Pneumocystis | 3 | 484 (671) | 66 |
Aspiration pneumonia | 51 | 239 (1932) | 68 |
ARDS, postoperative/trauma | 21 | 212 (711) | 66 |
ARDS, nonpostoperative/trauma | 238 | 377 (4013) | 65 |
Acute respiratory failure (non-ARDS) | 357 | 304 (7503) | 63 |
Other | 1686 | 274 (6011) | 60 |
ECMO provides adequate gas exchange and circulatory support without further exposure to high oxygen concentrations or high airway pressures, thus, fostering healing of the damaged lungs. The circulatory changes that result from initiation of ECMO also lower pulmonary vascular resistance. Draining right atrial blood reduces right atrial pressure and promotes closure of the foramen ovale. In addition, the reduced blood flow to the pulmonary vascular bed decreases pulmonary flow, reduces pulmonary artery pressure, and relieves right-to-left shunting through the patent ductus arteriosus. Well-oxygenated blood flowing left to right through the patent ductus arteriosus promotes its closure. By relieving hypoxia, hypercapnia, and acidosis, ECMO promotes relaxation of pulmonary vascular tone. The amount and extent of pulmonary arteriolar smooth muscle begins to regress. These changes allow the transition to a mature circulation. The infant continues to receive ECMO until parenchymal lung disease heals sufficiently to allow adequate gas exchange or fetal pulmonary artery flow converts to a mature state and adequate gas exchange occurs. However, pulmonary function tests indicate that infants are often weaned from ECMO with only moderate improvement in mechanical lung function. These observations support the impression that circulatory abnormalities contribute significantly to neonatal respiratory failure and may partially explain the difference in outcome between neonates and older patients treated with ECMO. Historically, neonates have had the best survival of all ECMO groups (>70%). In recent years, as more complex patients with multiple-organ failure have received ECMO, overall survival in neonates has shown some decline.
Infants with congenital diaphragmatic hernia comprise a special subgroup of patients treated with ECMO for severe pulmonary hypertension and respiratory failure. Severe pulmonary hypertension or lung hypoplasia leads to about 50% mortality even with ECMO support. Morphologic examination, better understanding of the pathophysiology of this lesion, and stabilization with ECMO as needed preoperatively or perioperatively has improved the survival rate in some centers in this challenging group of patients.
A 2009 study using Congenital Diaphragmatic Hernia (CDH) Study Group data showed that compared with repair on ECMO (44% survival), repair after ECMO showed a survival advantage (77% survival). The decision to repair on ECMO versus after the completion of ECMO is typically a choice by physician, institution, and ECMO team and subject to institutional bias.
Pediatric patients
Approximately 600 nonneonatal pediatric patients are reported to the ELSO registry each year for severe respiratory failure, with an overall survival rate of 53% (see Table 56.3 ). While pulmonary dysfunction resulting from bacterial or viral pneumonia, aspiration syndromes, intrapulmonary hemorrhage, and acute respiratory distress syndrome (ARDS) formed the majority of ECMO cases in the past, recent years have seen a large rise in less well-defined disorders recorded in the ELSO registry as “other.” This category may consist of patients with sickle cell disease, sepsis, and other diseases. The enormously heterogeneous older pediatric population spans nearly 2 decades of physiologic development, and cardiorespiratory failure develops as a result of a multitude of different disorders. Furthermore, many patients have varying degrees of multiple-organ failure along with respiratory disease at the time that ECMO is instituted. Resolution of both lung disease and secondary organ dysfunction must occur to achieve survival. These factors result in lower survival rates in older patients treated with ECMO than that achieved with the neonatal patient population.
One small subgroup of ECLS patients with excellent survival is individuals with status asthmaticus. One study of 64 asthmatic patients supported by ECLS noted that the mean duration of ECMO was 94 hours, and 94% of the patients survived. VV support was the cannulation mode in 86% of patients.
One common theme in pediatric ECMO over the past few years is the increasing complexity of patients. The multiple exclusion criteria used in the early days of ECMO have now been essentially eliminated, and each potential patient is generally considered on a case-by-case basis. Even patients with known bleeding disorders such as hemophilia have successfully received ECMO support. Between 1993 and 2007, the percentage of pediatric patients with comorbidities prior to ECMO increased from 18% to 47%. Overall, 40% to 50% survived to hospital discharge. Patients with pertussis and fungal pneumonia had the highest mortality; these groups remain among the most challenging ECMO patients. Of interest, pertussis is an exclusion diagnosis in some parts of Europe owing to the poor outcomes with ECMO. In some areas of the United States, however, pertussis ECMO patients have been treated successfully. One review of 200 pertussis patients who received ECMO found 28% survival, with leukocytosis (and leukoreduction) and pulmonary hypertension associated with poor outcome. Variability in strain of pertussis or response to infection may seem to be logical explanations for geographic variability in survival, but this has not been proven.
Trauma patients
Trauma patients, particularly those with multiple injuries, are at risk of respiratory failure. Trauma remains the leading cause of death in young adults. Common pathophysiologic mechanisms include direct chest injury-causing pulmonary contusion, long bone or pelvic fractures causing fat embolization, or an inflammatory-mediated event following systemic injury. ECMO provides “lung rest” by permitting reduced ventilator settings and limiting further ventilator-associated lung injury while maintaining tissue perfusion and oxygenation. Trauma patients have been previously excluded for consideration owing to their inherent high-risk bleeding. However, many centers have documented success in this patient population. In a study comprising 28 adult patients treated with ECMO because of trauma-related respiratory failure, 20 patients were successfully weaned off ECMO and discharged. Eight patients died as a result of overwhelming sepsis and irreversible cardiogenic shock. Good outcomes in pediatric patients undergoing ECMO following trauma also have been reported, including patients with severe thoracic trauma. , Traditionally, traumatic brain injury (TBI) patients were considered a relative contraindication to ECMO. However, more centers are describing successful use of ECMO in patients with TBI, even with recent ICH or with intracranial pressure monitoring in place. Craniotomy on ECMO for high intracranial pressure has also been described and, in a 10-year review from the ELSO registry, 3 non-TBI children who underwent craniotomy on ECMO support were described. Two survived with minimal neurologic deficits.
Other trauma-related applications of ECMO include management of patients with extreme hypothermia who require gradual extracorporeal rewarming. The old adage that “you aren’t dead until you are warm and dead” in hypothermic arrest holds true—bypass techniques such as ECMO are now considered the standard of care in severe hypothermic arrest resuscitation. Multiple “miracle” stories of survival with good neurologic function exist based on ECMO support in hypothermia. Trauma patients with massive hemorrhage and ongoing coagulopathy from transfusion-related hypothermia also have received short-term extracorporeal support with bypass to facilitate rewarming to temperatures that help normalize the coagulation process. Once rewarming is achieved, cannulas are withdrawn.
Extracorporeal membrane oxygenation and high-risk diseases
Patients with underlying malignancy form an increasingly large percentage of ECMO support, rising from 0.3% to 1% of pediatric ECMO cases in the most recent review. Of 171 patients analyzed from 2008 to 2012, 55.0% survived their ECLS run and 48.0% of patients survived to hospital discharge. Recent anecdotal reports have noted that patients with newly discovered malignancy have also received successful induction therapy while on ECMO support.
The most discouraging group of patients who have received ECMO are those with underlying bone marrow transplants. Although ECLS has been applied to such children, none have been reported as survivors to discharge in the ELSO registry. However, anecdotal reports of sporadic survival—combined with pleas from oncologists that the rapid rise of stem cell transplants and today’s conditioning and posttransplant regimens are less toxic, lead to rapid engraftment, and result in cure if the patient can be supported until engraftment occurs—result in application of ECMO in some patients. Most patients receive ECMO for respiratory failure (often of unknown etiology) or sepsis.
The effect of high-risk diagnoses on outcomes with ECMO support is highlighted by the recent evaluation of such patients via the Pediatric Health Information System. High risk was defined as diagnoses of bone marrow transplant, leukemia, lymphoma, neutropenia, immune system abnormalities, genetic abnormalities, neoplastic disorders, and complex congenital heart disease (CHD). Bone marrow transplant (81% mortality; OR, 3.49), leukemia (66% mortality; OR 1.88), and neutropenia (58% mortality; OR, 1.62) were associated with higher odds of mortality compared with other pediatric ECMO patients. Complex CHD (52% mortality) and genetic syndromes (48%) had no association with outcomes. ,
New therapies, such as chimeric antigen receptor (CAR) T-cell replacement for malignancy and likely soon for nonmalignant conditions in the future, are often associated with severe systemic inflammatory responses that are short-lived. Use of ECMO in such patients has provided support until this phase of treatment response is over.
Poisonings
ECMO has also provided respiratory and/or cardiac support for patients who have ingested toxic substances or overdosed. Calcium channel blockers, tricyclic antidepressants, β-blockers, aluminum phosphate, and a variety of other medications have all been successfully treated with ECMO. Use of ECMO should be related to the probability that temporary support can allow return of organ function and not necessarily related to clinical presentation, as some of these patients will have manifestations of fixed and dilated pupils, profound cardiac dysfunction to the point of asystole, and features that may seem incompatible with life but, in reality, are consistent with drug toxicity. An animal study of resuscitation with ECMO versus conventional therapy in severe carbon monoxide poisoning also found improved survival with ECMO rescue.
Bridge to lung transplant
One special patient population that is most applicable to adults but also to adolescents and even to younger patients is the use of ECMO as a bridge to transplant. With the advent of new equipment and patient management techniques that allow patients to be awake, mobile, and undergo active rehabilitation while on ECMO support, this area represents a new growth region for which excellent results are being reported in both pre- and posttransplant care. Especially in patients with severe hypercarbia, such as chronic obstructive pulmonary disease or cystic fibrosis, ECMO can provide an improved quality of life until transplant occurs. The artificial lung is also coming closer to clinical reality and may also offer support.
Why use extracorporeal membrane oxygenation?
ECMO avoids the circulatory derangements that often result from extreme forms of mechanical ventilation and provides systemic perfusion without the need for high-dose levels of inotropic agents. Thus, ECMO may prevent secondary organ dysfunction. While it is desirable to apply ECMO before multiple-organ failure has occurred, many patients already have secondary organ damage prior to ECMO. While survival in such patients is less than with single-organ failure, success can be obtained. In addition to providing D o 2 and circulatory support, ECMO provides a platform to which other organ support devices can be integrated. Renal replacement therapy, plasma exchange, and a variety of new filters that may absorb cytokines or provide hepatic support are able to be added as adjuncts to the ECMO circuit without adversely affecting the patient’s hemodynamics. ,
Extracorporeal membrane oxygenation for cardiac dysfunction
Details on extracorporeal support in cardiac patients are discussed in Chapter 28 .
Extracorporeal membrane oxygenation for resuscitation
Another growing area of the use of ECLS is in support of patients with refractory cardiac arrest. This type of support is termed extracorporeal cardiopulmonary resuscitation (ECPR), or ECMO during cardiac arrest. Many centers maintain an ECMO circuit setup that is preprimed with a crystalloid solution and stored (usually up to 30 days). This is done to facilitate expedient access to ECMO support for ECPR in situations of acute deterioration or whenever there is insufficient time or personnel for routine ECMO. Other centers use a portable centrifugal bypass perfusion system that also is easily set up within 10 minutes. Both methods use a hollow-fiber membrane lung.
An addendum to the ELSO registry that has been specifically designed for patients experiencing cardiac arrest may provide more detailed information to answer ongoing questions.
Several adult reports, which may have applicability to larger pediatric patients, have found that percutaneous femoral artery and vein cannulation during arrest is efficient and associated with good outcomes. Resuscitation efforts even in the field are now also being evaluated, with center ECMO teams or emergency medical personnel placing cannulas and initiating ECMO on-site. This process is aimed predominantly at those experiencing acute heart failure with ventricular fibrillation; whether this will result in improved outcomes is yet unknown. How these efforts may expand to children is unclear.
Extracorporeal membrane oxygenation and septic shock
The role of ECMO in severe septic shock is debatable and results vary. Patients with cardiomyopathy and vasoconstrictive shock induced by sepsis (so-called cold shock ) fare better than those with vasoplegia ( warm shock ). The most recent international guidelines for the management of septic shock in children specify a grade 2 recommendation for ECMO as a therapy of last resort for refractory septic shock. An international retrospective cohort study using data of children admitted to the intensive care unit (ICU) with a diagnosis of severe septic shock between 2006 and 2014 showed survival benefit in pediatric patients treated with ECMO for septic shock. Of the 164 patients who met the criteria for severe septic shock, survival to discharge was 40% in the conventional therapy group versus 50% in the ECMO group. In children who experienced an in-hospital cardiac arrest, survival to hospital discharge was 18% with conventional therapy versus 42% with VA ECMO. This article also reviewed primary outcome by level of mechanical support; survival was significantly higher in patients who received high ECMO flows (>150 mL/kg per minute 4 hours after institution of support) compared with children that received standard ECMO flows or no ECMO flow (82% vs. 48% and 42%, respectively). Patients with central cannulation had higher flow rates and improved survival when compared with those with peripheral cannulation.
Patient selection criteria
Various mortality prediction criteria have been put forth as indicators of when ECMO rescue is best applied. Many of these criteria have been derived from small series of historical data for patients with respiratory failure or were extrapolated from neonatal respiratory failure data. Attempts to provide universally accepted criteria for the institution of ECMO have proved to be difficult. New severity scores and outcome predictors for ECMO have been developed, although none is perfect. The respiratory ECMO survival prediction score, the predicting death for severe acute respiratory distress syndrome on VV-ECMO score, the pediatric pulmonary rescue with ECMO prediction score, the pediatric ECMO prediction score, and the pediatric risk estimate score for children using extracorporeal respiratory support all have some potential, but further refinement is needed ( Table 56.5 ). They are most useful to provide families and clinicians with some general outcome prediction and not for use in individual patient ECMO selection currently. Online calculators are available for some of these scores and can be accessed via the ELSO website or from web addresses within the specific publications.
Core | Population | No. Variables | Model ROC | Development Dataset | External Validation | Website | Reference |
---|---|---|---|---|---|---|---|
PIPER | Neonatal respiratory failure | 8 | 0.74 (continuous); 0.73 (binned) | 2000–2010 | No | https://www.elso.org/Resources/PIPERScore.aspx | 174 |
Neo-RESCUERS | Neonatal respiratory failure | 10 | 0.78 | 2008–2013 | No | http://www.neo-rescuers.com | 175 |
Ped-Rescuers | Pediatric respiratory failure | 13 | 0.69 | 2009–2014 | No | http://www.ped-rescuers.com | 176 |
P-PREP | Pediatric respiratory failure | 6 | 0.69 | 2001–2013 | Yes | http://www.picuscientist.org/pprep | 177 |
RESP | Adult respiratory failure | 12 | 0.74 | 2000–2012 | Yes | http://www.respscore.com | 178 |
PEP | Neo and Peds ECMOAll categories | 8 | 0.75 | 2012–2014 | No; pending | https://www.cpccrn.org/calculators/ecmoprediction/ | 179 |
SAVE | Adult cardiogenic shock | 11 | 0.68 | 2003–2013 | Yes | https://www.elso.org/SaveScore/Index.html | 180 |
CDH Pre-ECMO | Neonates with congenital diaphragmatic hernia | 12 | 0.65 | 2000–2015 | No | https://www.choc.org/ecmocalc/ | 181 |
CDH On-ECMO | 22 | 0.73 |
The current state is that almost every patient who receives ECLS is selected on a case-by-case basis. The clinical team discusses the risks and benefits of ECLS in light of the current ICU status and makes a decision to either offer ECMO to the family as an option or not. Given the complexity of patients receiving ECLS and comorbidities often present, clinicians from other services outside the ICU staff (pulmonary, oncology, neurology, and others) are beneficial in this discussion. Many centers also have smaller teams with special expertise or interest in ECLS within the overall ICU faculty who function to provide advice on candidacy, ECLS strategy, and patient management.
For the majority of patients who undergo ECMO, less invasive methods of respiratory support have failed. Such methods of support often include conventional mechanical ventilation in pressure control or pressure-regulated volume control modes, high positive end-expiratory pressure (PEEP), high-frequency ventilation, surfactant, inhaled nitric oxide, prone positioning, and neuromuscular blockade.
While severity scores such as partial pressure of arterial oxygen/fraction of inspired oxygen (Pa o 2 /Fi o 2 ), shunt fraction, compliance and others have been used to identify potential ECMO candidates, the oxygenation index (OI) has remained in favor in children, as it combines the level of ventilatory support being given and the oxygen level obtained in arterial blood. It is calculated as follows:
OI=Mean Airway Pressure (cm H2O)×FiO2PaO2(torr)
Scores of greater than 40 or from 30 to 40 without improvement have been associated with high mortality in the past and had been used as ECMO candidacy alerts. Recent data, however, have noted doubling of mortality from less than 20% to more than 40% when the OI exceeds 16. In an evaluation of 65 children with serial OI measurements obtained from the electronic medical record from a single center, mortality tripled when the OI exceeded 17. In another review of factors associated with death in children with severe respiratory failure, the peak OI and pediatric risk of mortality score were found to be independent predictors of outcome, although no definitive OI cutoff that predicted death could be identified. These findings, along with the increased ease of applying ECMO, have led to discussion as to whether ECMO should be applied at OI severity scores much lower than in the past. Indeed, in the adult population, there is now discussion as to whether ECMO should be implemented to avert intubation or if it should be performed shortly after the need for mechanical ventilation. , Alveolar dead space has also been linked to outcome, with one study noting that dead space of 30% or greater at the onset of pediatric ARDS differentiated survivors from nonsurvivors. The impact of dead space was not significant after 24 hours.
In an attempt to provide standard definitions for respiratory failure in children and develop consensus regarding pediatric respiratory care guidelines, an expert panel of 19 international clinicians met and examined thousands of published reports by the Rand/University of California, Los Angeles (UCLA) method and achieved consensus results using the modified Delphi method. ECLS was one of the nine areas selected for recommendations. As there were few high-quality report scores for ECMO as scored by the Rand/UCLA method, recommendations were developed primarily by consensus expert opinion. These included the following:
- 1.
Serial measurements of severity of illness as criteria for ECMO should be used rather than a single cut-off value.
- 2.
Cases should be discussed among treating clinicians as a team.
- 3.
ECMO should not be offered to patients in whom life-sustaining measures were restricted.
- 4.
Quality of life and long-term outcomes from comorbidities should also be evaluated.
- 5.
All centers should report patients and outcome to a registry such as ELSO.
- 6.
Benchmarking of centers against overall data from such registries is useful to improve quality care.
This consensus report represents the baseline agreement on respiratory definitions and care practices. It is likely that further refinement of recommendations will occur over time.
Physiology of extracorporeal life support: Gas exchange and oxygen delivery
Oxygenation
The difference between the partial pressure of oxygen (P o 2 ) in the gas supplied to the oxygenator and that in the patient’s systemic venous blood provides the “driving pressure” across the membrane lung. As an example, 30% oxygen blended into the gas entering the oxygenator will result in an estimated Pa o 2 of about 228 torr at sea level. The P o 2 of venous blood entering the oxygenator depends on the difference between D o 2 and consumption in the patient but is about 40 torr in normal conditions. Thus, the driving pressure for oxygen diffusion into the blood would be approximately 188 torr (228 torr − 40 torr = 188 torr), which is adequate to achieve 100% saturation of hemoglobin. Higher oxygen concentrations in the gas phase may be necessary to compensate for the loss of membrane surface area over time to maintain hemoglobin saturation. Faster flows through the oxygenator may also decrease the time for gas exchange to occur. At postoxygenator oxygen saturations greater than 95%, increasing the oxygen concentration of the sweep gas has little incremental effect on blood oxygen content (remember that the contribution of oxygen content by the Pa o 2 is small compared with the effect of hemoglobin and oxygen saturation). For this reason, oxygen concentration in sweep gas usually is adjusted to maintain an oxygen saturation of approximately 95% in postoxygenator blood. While providing the most oxygen to the patient would seem to be beneficial, there are now several reports associating high levels of Pa o 2 returning to the patient with adverse events. Whether these represent poor cardiac function so that the majority of D o 2 is via the ECMO circuit without native venous admixture (and profound cardiac failure may have a higher risk of death), induction of oxygen radicals or other factors has not been proven. Of interest, however, recent data have also identified higher levels of oxygenation in adult respiratory failure patients with decreased survival as compared with those with lower levels of measured Pa o 2 . Similar to now resuscitating newborns with room air, ECMO initiation may be changing from high levels of Fi o 2 to the membrane lung to those that just maintain postmembrane saturations of 95% to 100%.
Carbon dioxide exchange
Even though the pressure gradient for CO 2 between venous blood and membrane gas is less than that for oxygen, CO 2 removal is excellent across the membrane lung. Despite the small pressure difference, the membrane’s high diffusion coefficient for CO 2 allows excellent CO 2 removal, even at low flow rates. To eliminate more CO 2 , the gas flow in the membrane must be increased, much as alveolar ventilation must increase to eliminate CO 2 from the body under physiologic conditions. CO 2 removal is also limited by the surface area across which gas exchange can occur. Thus, increased CO 2 clearance may be obtained by using larger oxygenators or using more than one oxygenator in parallel in the circuit. Conversely, to prevent excessive CO 2 removal and hypocapnia in small infants and neonates, the sweep of gas through the membrane lung can be reduced or low levels of CO 2 may be blended into the gas mixture to further reduce the partial pressure difference between blood and gas and maintain normocarbia.
Oxygen delivery
During VA ECMO for respiratory failure, both increasing D o 2 and increasing the patient’s Pa o 2 and arterial saturation can be accomplished by increasing the ECMO flow rate (“if the O’s are low, increase the flow”). This strategy diverts more of the systemic venous return into the ECMO circuit for oxygenation while at the same time proportionally decreasing the amount of venous blood that enters the diseased pulmonary circuit. Studies have suggested that complete bypass of the pulmonary circuit may lead to pulmonary alkalosis or ischemia and cause direct damage to the pulmonary capillary bed. Microsphere studies have shown that the majority of coronary artery perfusion during VA ECMO comes from native left heart ejection, which is another reason why ECMO is performed in a partial bypass state. , The result of increasing ECMO flow will be an increase in D o 2 provided by the circuit and an elevation in measured systemic arterial saturation and Pa o 2 . Another means to change the proportion of native blood flow to that from the ECMO circuit is to decrease the overall blood volume in the patient (not usually done). During cardiopulmonary bypass, filling pressures and overall blood volume can be adjusted by removal of blood volume into the bypass circuit and venous reservoir. During ECMO, these same principles can be followed: excessively high filling pressures can be lowered by simple removal of blood volume from the circuit (not usually done), and diuretics and renal replacement strategies can be used to control fluid balance. However, care must be taken to avoid decreasing circulating volume excessively—this may, in turn, cause tissue hypoperfusion or an increase in oxygen extraction.
As blood returning from the ECMO circuit is already highly saturated with oxygen, oxygen content can also be augmented by increasing hemoglobin levels in the patient. D o 2 supplied by the ECMO circuit can be calculated by pump flow as the surrogate for cardiac output and oxygen content by hemoglobin and postmembrane lung oxygen saturation. Determining the amount of D o 2 supplied by the native heart is less clearly calculated. Monitoring of adequate D o 2 is aided by following venous saturation and other markers, such as lactate, perfusion, urine output, and mental status. If ECMO flow cannot be increased enough to provide adequate support, an additional drainage cannula to provide more venous return and augment ECMO flow may be needed. In centrifugal pump ECMO, reduction of mean arterial blood pressure in the patient may also allow easier forward flow from the centrifugal circuit and improve D o 2 .
Physiologic equations applied to ECMO may help identify adequacy of support:
DO2=Blood flow (L)×(CaO2)×10
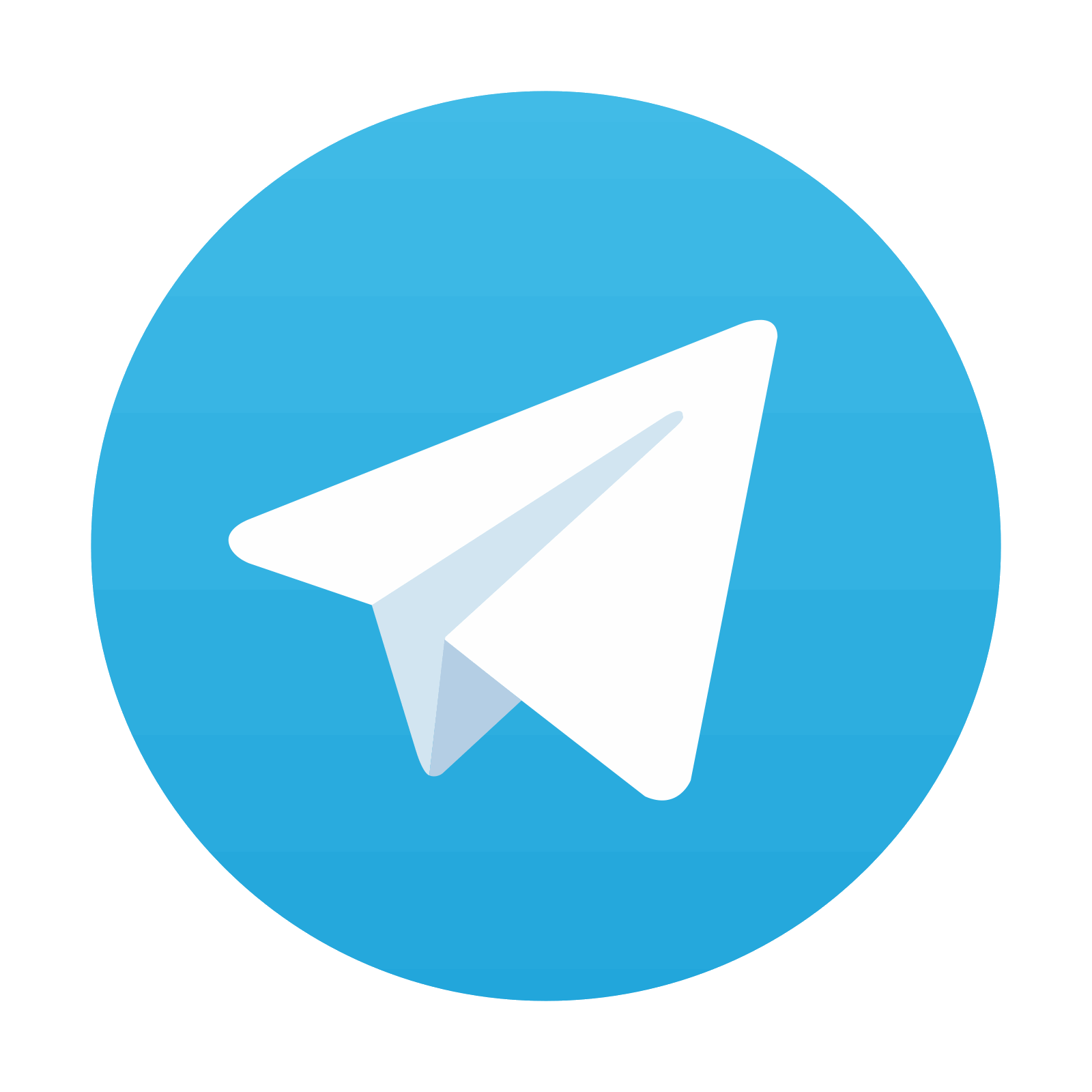
Stay updated, free articles. Join our Telegram channel
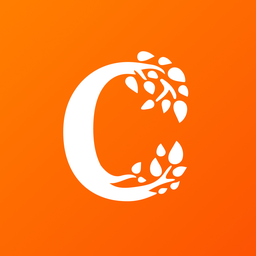
Full access? Get Clinical Tree
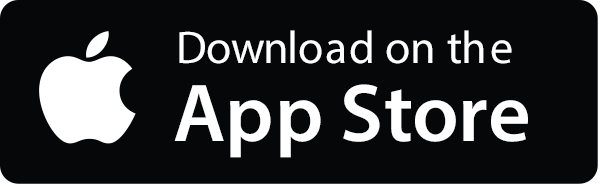
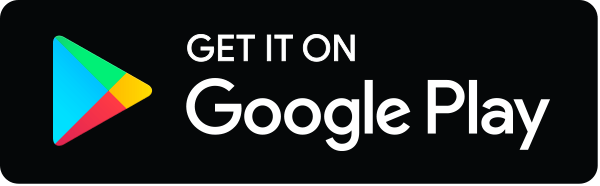
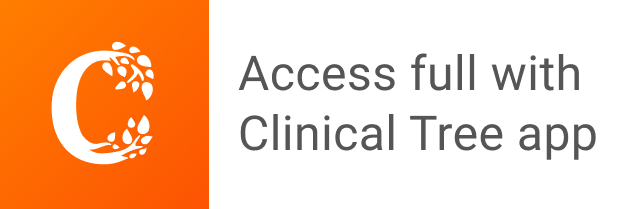