Pearls
- •
The intensive care unit is a unique environment for performing an echocardiogram; the intensivist, sonographer, and pediatric cardiologist must work together to obtain the needed information with minimal impact to the patient.
- •
The main components of the pediatric echocardiogram are two-dimensional imaging, color Doppler, and spectral Doppler. Tissue Doppler, three-dimensional imaging, and strain analysis are additional components that supplement the typical examination.
- •
Cardiac point-of-care ultrasound provides an opportunity for rapid assessment of specific cardiac findings. However, like transthoracic echocardiography, it is highly dependent on the technology used and expertise. Many measurements standardized for use in adult patients have not been fully validated in children with or without congenital heart disease.
- •
Evaluation of volume status should include the assessment of multiple rather than single measurements and must be placed into the clinical context (e.g., baseline heart disease and physiology, intervention performed, cardiac rhythm).
- •
Echocardiography can provide a noninvasive quantitative and qualitative estimate of pulmonary artery and right ventricular systolic pressures.
- •
Pericardial effusion can lead to hemodynamic compromise. Echocardiography is an essential tool for the assessment of pericardial effusion and can be used to guide percutaneous drainage.
- •
Pediatric patients presenting with new-onset heart failure should have echocardiographic assessment of coronary artery origins. Echocardiography can diagnose most coronary artery abnormalities; however, in some patients, angiographic evaluation is necessary.
Patients in the pediatric intensive care unit (ICU) often have hemodynamic or cardiac instability. This can occur in the setting of congenital and acquired cardiovascular disease. The pediatric intensivist must be aware of these disease states and be proficient in the assessment of cardiovascular status. Multiple tools can be used in this assessment, including physical examination, vital signs monitoring, intravascular lines, near-infrared spectrometry, and acid-base analysis. An additional tool is echocardiography, which can be invaluable in supporting the clinical evaluation of the hemodynamically compromised patient.
Echocardiography is the use of ultrasound to image cardiovascular structures. The echocardiography probe transmits sound waves through the body and receives the reflections of these sound waves; the processor uses the data to generate an image. Echocardiography arose as a widespread clinical diagnostic test in the 1970s and 1980s. Previously, invasive angiography was the cardiac diagnostic test of choice. Echocardiography supplanted invasive angiography because of its ability to obtain anatomic, hemodynamic, and functional data noninvasively. Additional advantages of echocardiography include the absence of ionizing radiation, its portable nature, and the fact that most patients do not require sedation/anesthesia. Limitations include the long time it takes to perform a complete study, poor image quality in patients with greater mass, the fact that some hemodynamic and functional assessments are indirect measurements or calculations, and the need for a highly skilled operator. These strengths and weaknesses highlight both the usefulness of echocardiography in the ICU setting and the importance of interpreting results with the knowledge of its limitations.
Performing an echocardiogram in the ICU is unique from the examination in the traditional echocardiography laboratory. The critical nature of the patient often requires that the study be performed at the bedside. Thus, the cardiac sonographer and echocardiography machine must travel to the patient. The intensivist, cardiac sonographer, and pediatric cardiologist should have a preliminary conversation about the goals of the study, patient stability, and need for sedation. The goal is always to maximize clinical information with minimal disturbance of the patient. Sedation may be required to minimize patient agitation and instability. The ICU team should work with the cardiac sonographer and pediatric cardiologist to ensure proper sedation and monitoring. A complete pediatric echocardiogram is the preferred goal. However, at times, a limited study with a focused clinical question can be considered in order to minimize the impact on patient stability. Following image acquisition and interpretation, the ICU team and the pediatric cardiologist should discuss the results in the context of the patient’s clinical status.
Components of the examination
An echocardiography evaluation consists of multiple tools. Two-dimensional (2D) echocardiography is the backbone of the study. It provides images in a still frame or video format, allowing assessment of anatomic details, valve movement, and ventricular wall motion. Color Doppler images can be superimposed on 2D images to provide information about direction and velocity of blood flow. Color Doppler images reveal critical information about valve function (stenosis and regurgitation) and blood flow through the cardiovascular system. Spectral Doppler builds on color Doppler, allowing for quantification of velocity in a specific vector. Velocity can be graphed as a function of time and the Doppler signal pattern provides important hemodynamic information ( Fig. 29.1 ). The velocity can be measured and entered into the modified Bernoulli equation to determine peak or mean gradients across valves or vessels.

The accuracy of spectral Doppler can be limited by poor alignment of the vector to the direction of blood flow and improper probe selection. Spectral Doppler gradients may not always match gradients measured at cardiac catheterization. This can be true because of a number of variables—such as sedation status, peak instantaneous gradients versus peak-to-peak gradients, and pressure recovery phenomena. , These echocardiographic measurements must be interpreted in the setting of other clinical and echocardiographic findings. 2D echocardiography, color Doppler, and spectral Doppler are the primary components of the pediatric echocardiogram.
Additional components of a pediatric echocardiogram include tissue Doppler, three-dimensional (3D) imaging, and strain analysis. Tissue Doppler measures the velocity of the myocardium at the level of the atrioventricular valves. The velocities and the timing of myocardial motion provide important information about diastolic ventricular function and electrical-mechanical coupling. , 3D imaging can provide important ancillary information, including quantification of left ventricular systolic function ( Fig. 29.2 ). 3D imaging can also provide high-quality anatomic information, particularly regarding atrioventricular valves. Strain imaging evaluates the deformation of myocardium and can provide information about regional and global systolic function, allowing for the detection of subclinical changes in ventricular function. ,

Modalities
Transthoracic echocardiography
Transthoracic echocardiography is the clinical workhorse of pediatric cardiac imaging. This consists of placing the probe directly on the patient’s chest wall and abdomen and transmitting/receiving ultrasound. Its noninvasive nature makes it a readily available imaging modality with minimal risk. Image spatial resolution and quality are improved with high-frequency probes; however, high-frequency probes have limited penetration. Higher-frequency probes can generate images of excellent quality in patients with smaller mass, such as young children. Patients with greater mass require lower-frequency probes with increased penetration; however, image quality and spatial resolution are sacrificed. Any material between the probe and the patient—such as bandages, electrocardiography (ECG) leads, chest tubes, and pacing wires–can limit transthoracic echocardiography image quality.
Transesophageal echocardiography
Transesophageal echocardiography (TEE) involves placing a specialized TEE probe through the patient’s oropharynx into the esophagus and stomach, allowing for superior visualization of intracardiac structures. TEE is somewhat limited in the visualization of extracardiac structures such as the aortic arch, branch pulmonary arteries, and distal systemic and pulmonary veins. TEE requires sedation. Thus, when performed in the ICU, it requires assistance by the critical care or anesthesia team. Sedation should be sufficient to provide appropriate analgesia and amnesia. It should also keep the patient motionless for safety and better image quality. Risks, in addition to sedation, include bleeding and injury to the oropharynx, teeth, esophagus, and stomach.
Common indications for a TEE in the ICU setting include poor transthoracic echocardiography image quality, endocarditis, and intracardiac thrombus in the setting of embolic phenomena or persistent atrial arrhythmias. The identification of a vegetation on cardiac imaging is a major criterion in the modified Duke criteria for endocarditis. TEE is more sensitive than transthoracic echocardiography and is the gold standard for detecting vegetations. , However, TEE can have limited sensitivity in some patients, necessitating additional imaging modalities. Periprocedural TEEs, such as those performed during congenital cardiac surgery, allow for procedural guidance and postoperative anatomic and functional assessment. In the pediatric cardiothoracic operating room, the postoperative TEE may be complemented by an epicardial echocardiogram. This involves placing a transthoracic probe in a sterile sleeve that is then positioned directly onto the cardiac surface. Proper interpretation of periprocedural TEE and epicardial echocardiogram is critical to the postprocedural care of these patients.
Fetal echocardiography
Fetal echocardiography involves the evaluation of fetal cardiovascular anatomy and function. These studies are performed by transabdominal imaging, although transvaginal studies can also be performed. It would be unusual for a pediatric ICU patient to require a fetal echocardiogram, but the mother of a neonate in the pediatric ICU may have had a fetal echocardiogram during pregnancy ( Fig. 29.3 ). Therefore, the intensivist should be familiar with the interpretation of these results. Currently, many patients with congenital heart disease have a prenatal diagnosis. Fetal echocardiography has a 68% sensitivity for detecting congenital heart disease ; however, it has some limitations. Standard postnatal congenital heart disease screening (physical examination and pulse oximeter) should be performed even in the patient with a normal fetal echocardiogram. A prenatal diagnosis allows for proper planning leading up to the delivery and postnatal care. Fetal echocardiography can also guide intrauterine therapies, such as transplacental pharmacologic treatment of fetal arrhythmias, fetal balloon atrial septostomy, and aortic balloon valvuloplasty. Fetal echocardiography has been shown to improve outcomes in some congenital heart disease populations. A proper fetal diagnosis can also alleviate unnecessary postnatal anxiety and inform decisions regarding safe maternal-infant bonding in the delivery room.

Intracardiac echocardiography
Intracardiac echocardiography (ICE) involves the transvenous insertion of a specialized echocardiography probe into cardiac chambers. This allows for detailed imaging with the probe in very close proximity to intracardiac structures. The obvious risk of this approach is its invasive nature and need for transvenous access. Because of this, ICE is predominantly performed in the cardiac catheterization laboratory where adequate transvenous access is already obtained. In this setting, ICE can be used to guide the transvenous placement of intracardiac devices. ICE has limited application in the pediatric ICU setting; however, the intensivist caring for patients after cardiac catheterization should be familiar with this imaging modality and at least have a cursory understanding of its features.
Cross-sectional imaging
Cross-sectional imaging modalities, such as cardiovascular magnetic resonance imaging (CMR) and cardiac computed tomography (CT), may be a useful adjunct to echocardiography in some pediatric ICU patients. These modalities can provide important additional information, particularly when delineating extracardiac vascular structures. Cardiac CT provides excellent spatial resolution, which is most useful in small patients and when imaging small structures, such as coronary arteries. An additional advantage of cardiac CT is its short image acquisition (scanning) time. Its disadvantages include lack of portability (stationary location), nephrotoxicity of intravenous iodinated contrast, and radiation exposure with subsequent increased malignancy risk. CMR offers the advantages of myocardial tissue characterization and is an excellent imaging modality in the evaluation of the pediatric patient with elevated troponins and possible myocarditis. CMR also allows for accurate quantification of ventricular volumes, which may be instrumental in the surgical planning of patients with hypoplastic ventricles or regurgitant valves. The use of CMR is limited by its long imaging time, need for sedation/anesthesia in younger patients, stationary location, limited use in patients with renal failure, and contraindications to metal support devices in the scanner.
Point-of-care ultrasound
Point-of-care ultrasound (POCUS) represents a balance between quick access to simplified scanning technology involving the fewest people and the limitations imposed by potentially reduced machine functionality coupled with greater disease complexity. Its applications range from assessment of cardiac function and intravascular volume status to evaluations of abdominal processes, pulmonary disease, bladder filling, and rapid trauma assessment. Cardiac POCUS is now routinely used in critical care, emergency and hospital medicine, and veterinary practices along with the neonatal ICU environment, with the latter use often termed targeted neonatal echocardiography . Training in cardiac POCUS has been advocated to begin in medical or allied health professions schools, with different approaches ranging from an extension of the physical examination to a limited diagnostic study in its own right. Guidelines and standards for training and its use in adult patients have been published by echocardiography societies and other national organizations.
The use of POCUS in children has paralleled its increased application in adults, including the recent publication of evidence-based guidelines for its use in critically ill children and infants. Several considerations are necessary for the expansion of cardiac POCUS use in pediatrics. These include adapting scanning equipment designed primarily for adults to the different needs of children, such as higher-frequency transducers and more frequently displayed frame rates for faster heart rates, plus establishing additional training and appropriate usage criteria for children. Not all accepted cardiac measurement techniques for adults have been fully validated in children or in the setting of congenital heart disease. Consequently, the patient’s underlying status should drive the decision on whether the strengths of a fast cardiac POCUS assessment of ventricular function will benefit the patient (i.e., if the patient has a systemic left ventricle) or will be potentially confounded by abnormal anatomy and physiology (i.e., a patient with a single right ventricle and a Fontan, or cardiac malposition). Most importantly, any obtained images should be archived for comparison to guide future studies and for quality assurance. Cardiac POCUS has been evaluated in the pediatric ICU and has been found to be sensitive for assessment of pericardial effusion and left ventricular size and function. However, benefits beyond these have not been proven.
Structural congenital heart disease and intracardiac shunting
Transthoracic echocardiography is the test of choice for accurately diagnosing most congenital heart disease in pediatric patients. In the ICU setting, an initial limited echocardiographic evaluation can help guide therapy for new patients by showing obstruction to blood flow, assessing ventricular and valvar function, and demonstrating the source of both pulmonary and systemic blood flow. This allows for a rapid assessment of the patient’s physiologic state and the need for therapies, such as a prostaglandin infusion for ductal patency or inotropic support. After initial stabilization, performance of a complete echocardiogram is essential to evaluate the details of cardiovascular anatomy for surgical or other interventional planning.
The evaluation of cardiac shunting and blood flow is an essential component in the assessment of cardiovascular physiology. At the atrial level, reversal of the normal left-to-right shunt is indicative of an elevation in right atrial pressure above left atrial pressure, which can be seen in situations such as tricuspid valve stenosis, right ventricular dysfunction, or pulmonary hypertension. An elevation in the mean pressure gradient of an atrial shunt can be seen in single-ventricle patients with a restrictive atrial septum ( Figs. 29.4 and 29.5 ), hemodynamically significant mitral or tricuspid stenosis with inadequate forward flow, or in cardiomyopathy with significantly elevated ventricular filling pressures. Ventricular shunt direction can be used to evaluate the difference in ventricular pressures. In the setting of unrepaired tetralogy of Fallot, the reversal of normal left-to-right ventricular shunt can be seen in the setting of a hypercyanotic spell caused by right ventricular outflow tract obstruction. In the absence of aortic obstruction, the left ventricular systolic blood pressure is the same as the systemic systolic blood pressure. Therefore, a ventricular shunt gradient can be used to estimate the right ventricular systolic pressure. In patients with aortic or pulmonary obstruction, the direction of shunt in the ductus arteriosus can give an important clue as to the hemodynamic significance of the stenosis. If there is inadequate forward aortic or pulmonary flow, then ductal flow is in the direction to augment that flow. In the absence of left or right ventricular outflow tract obstruction, the direction and velocity of shunting across a restrictive patent ductus arteriosus can provide an estimate of pulmonary artery pressures.


Valve anatomy and function
Assessment of valve function is an important part of the evaluation of cardiovascular physiology in the ICU. 2D imaging of valve structure provides information about valve anatomy that is important in surgical planning, determining the mechanisms for valve dysfunction, and evaluating for acquired diseases such as endocarditis ( Fig. 29.6 ). Color flow Doppler can show the location and size of regurgitation jets and flow turbulence in areas of abnormal flow, such as valve stenosis. Spectral Doppler tracings allow for quantification of valve stenosis by measurement of peak and mean gradients across valves. For measurement of peak gradients, the simplified Bernoulli equation (provided earlier) is used to accurately estimate pressure. The mean pressure gradient is calculated from a tracing of the spectral Doppler velocity curve.

Atrioventricular valve regurgitation and stenosis are important factors in ventricular filling and function. The evaluation of regurgitation is difficult, as there are no reliable quantifying measures in the congenital cardiac population, in which the valve anatomy is often abnormal and there are frequently multiple regurgitant jets. Assessment of atrioventricular valve regurgitation is aided by looking at secondary changes, such as atrial enlargement and pulmonary or systemic vein flow reversal with ventricular systole. Therefore, the best assessment of atrioventricular valve regurgitation is by the subjective judgment of a trained observer who can take into account all of these factors. The peak atrioventricular valve spectral Doppler gradient is used to estimate systolic ventricular pressure by adding it to the directly measured or assumed associated atrial pressure. This is used for the assessment of pulmonary ventricular or pulmonary artery hypertension. The peak gradient of systemic atrioventricular valve regurgitation can also be used to estimate systemic ventricular pressure. The mean gradient across an atrioventricular valve correlates well with direct measurements by cardiac catheterization, whereas the peak gradient is much less useful. Secondary findings with significant atrioventricular valve stenosis include atrial enlargement and systemic or pulmonary vein flow reversal with atrial systole.
Semilunar valve function directly affects the effective cardiac output to the pulmonary and systemic circulations. Both semilunar valve stenosis and regurgitation affect myocardial oxygen demand by increasing the pressure and volume load, respectively, on the associated ventricle. Regurgitation is best quantified by the width of the regurgitant jet, as the length of the jet and deceleration slope are affected by the pressure gradient across the valve and ventricular filling pressures. In the setting of more severe regurgitation, secondary flow reversal is seen in the pulmonary arteries and descending or abdominal aorta. The pulmonary regurgitation jet can be especially useful in the setting of pulmonary hypertension, as it allows measurement of the pulmonary artery end-diastolic pressure ( Fig. 29.7 ). The peak spectral Doppler gradient across the pulmonary valve correlates best with a direct peak-to-peak measurement by cardiac catheterization while the mean spectral Doppler gradient has a better correlation for the aortic valve ( Fig. 29.8 ).


Ventricular function
Assessing volume status
Any assessment of cardiac function must include an assessment of the loading conditions under which the heart is working. Most simply, these are preload (the filling status of the heart) and afterload (the resistance against which the heart pumps). With almost no exception, every measurement of cardiac systolic or diastolic function is affected by one or the other (or both) of these loading conditions within the range of expected human physiology.
Much has been written about the noninvasive assessment of volume status given the significance of right atrial pressures for right-sided hemodynamics and outcomes, and to guide decisions on fluid management. For instance, a child with a hypertrophied right ventricle that is stiff from cardiopulmonary bypass immediately after repair of tetralogy of Fallot may exhibit higher right atrial pressures with the amount of fluid needed to maintain right ventricular preload and cardiac output than a child with a surgically enlarged right atrium after heart transplantation performed with a biatrial anastomosis. Knowing when preload and fluid state are excessive and diuretics are required is similarly useful, as in the setting of pulmonary hypertension.
For adult patients, several guidelines and recommendations are available that reasonably predict right atrial pressure from noninvasive measurements, particularly involving inferior vena caval size and changes with respiratory maneuvers. Other measurements have included spectral Doppler inflow velocities and patterns, tissue Doppler measurements, , speckle tracking strain rates, and both 2D and 3D measurements of right atrial size. However, many of these measurements fail to achieve clinical utility when applied to critically ill adults with considerable overlap between fluid-responsive and non–fluid-responsive groups and poor interrater reliability. This creates a clinical “gray zone” in which measurements are helpful at the extremes but not helpful to guide more nuanced management. All of these problems are exacerbated in the acute postcardiothoracic surgery setting and lose correlation in the setting of positive-pressure ventilation.
The application of these techniques to children is even more problematic, especially in a critical care setting. Confounders can range from the simple, such as how to standardize a “sniff” in a child to assess inferior vena cava collapsibility, to the more complex, such as the physiologic impact of one patient with an intracardiac shunt compared with a second child without any shunt. Not surprisingly, conflicting data are reported in the literature. These likely reflect the differences in compliance of varying structures such as the right atrium, liver/inferior vena cava, abdomen, and chest in the pediatric patient compared with the adult, among other reasons. However, research in this area continues, with pediatric systemic venous and right atrial normograms now published, and simultaneous catheter-echo studies reported on the utility of advanced imaging techniques such as diastolic strain rate and the utility of the systolic:diastolic ratio.
Assessing diastolic function
The assessment of diastolic function is a natural extension of the assessment of volume status, as it incorporates the ventricular response to preload and filling, and atrial pressure reflects ventricular diastolic pressure. This is a topic of great interest in adult and pediatric cardiology—in particular for the systemic ventricle (typically, the left ventricle)—both due to its greater challenge in measurement and the significant impact that abnormalities in left ventricular diastolic function may have on clinical outcomes. , Right atrial pressure can be directly measured with relative ease through the placement of a central venous catheter. In contrast, left atrial pressure can only be indirectly measured via a pulmonary capillary wedge pressure, which involves increased risk to the patient, or directly via transseptal puncture and placement of a catheter into the left atrium (a higher-risk procedure both in placement and opportunity for systemic embolization of air or thrombus). Direct measurement of the left ventricular diastolic pressure is even more complicated, requiring either prograde passage of a transseptal catheter across the mitral valve or retrograde passage of a catheter across the aortic valve. All of these challenges make the noninvasive assessment of diastolic function more enticing and important.
In general, diastolic function can be affected by three different variables: impaired relaxation (early diastole), reduced restoring forces (recoil and untwisting), and decreased compliance/increased chamber stiffness (late diastole). These are assessed using multiple 2D, spectral Doppler, and tissue Doppler measurements. Common measurements include the mitral valve E/A wave Doppler ratio, E/e′ ratio, E wave deceleration time, left atrial size, mitral valve A wave duration, and pulmonary vein atrial systolic (Ar) wave reversal duration. Diastolic function grades, in both normal and diseased states, have been published for use in adults and are commonly employed in adult echo labs worldwide. However, as for volume status, these measurements are affected by cardiac rhythm, heart rate (which may fuse inflow Doppler waves), atrial anatomy, and need for precise positioning of the pulsed wave sample volume for spectral Doppler measurements. The use of multiple measurements is specifically recommended over use of a single measurement, as is inclusion of the standard vital signs, 2D imaging data, and clinical context. The most recent guidelines state that these measurements “are not necessarily applicable to children or the perioperative setting.”
This explicit caution about directly extrapolating adult diastolic guidelines to pediatrics has been proven reasonable in several publications. One of the first, in 2013, applied published adult diastolic guidelines to children with varying forms of cardiomyopathy, all of whom would be reasonably expected to have abnormal diastolic function. Use of the guidelines, however, failed to reliably distinguish diastolic function abnormalities between groups and demonstrated additionally poor interobserver agreement. Subsequent studies for the right ventricle and left ventricle have further demonstrated that, as compared with adults, children may not progress through the same stages of diastolic dysfunction and that early (impaired relaxation) and late (decreased compliance) abnormalities may overlap considerably in the same patient. , Challenges in connecting abnormal diastolic measurements to outcomes are also present in the setting of congenital heart disease, particularly in Fontan patients. ,
Reasons for this problematic extrapolation of adult guidelines to children are several, including maturational changes in the myocardium (age), loading conditions (affected by the variables discussed earlier under assessing volume status), ventricular geometry, atrioventricular valve function, and ventricular-ventricular interactions in addition to the standard confounders of heart rate and rhythm. Of these, maturational changes may be easily overlooked until one considers the fetus, which has classic evidence of diastolic dysfunction even in the healthy state due to the differences in loading conditions, pulmonary physiology, decreased compliance produced by the fluid-filled intrauterine environment, and a myocardium that is stiffer with fewer contractile elements.
Active research continues in this area despite these challenges and with the understanding that novel approaches may be needed. These include investigation into systolic/diastolic coupling and twist/untwist relationships, use of advanced imaging techniques such as diastolic strain rate measurements, or consideration of focused assessment on atrial mechanics, and its reservoir, conduit, and contractile functional components. , These investigations may occur separately or in parallel to adult investigations, with the crucial understanding that careful validation is necessary before any clinical extrapolation is made between the two groups.
Assessing systolic function
Assessment of systolic ventricular function is one of the most common reasons for obtaining an echocardiogram in the ICU setting. It is also important to remember that ventricular function is dependent on contractility, or ability to generate force at the level of the myocyte, as well as by pump function, or the deformation of the chamber leading to cardiac output. Ventricular function is affected by loading conditions, such as volume status, valve function and blood pressure, and by inotropic support ( Fig. 29.9 ). At times, wall motion may appear decreased despite normal contractility. Conversely, wall function can appear to be normal despite decreased contractility, such as in a patient with decreased function but that responds to inotropic support and an afterload-reducing agent. Additionally, congenital heart defects may distort the normal ventricular anatomy, which may affect accuracy of some of the standard methods of assessing ventricular function. A simple assessment of wall motion does not provide a complete picture of ventricular function.

Left ventricular systolic function
Left ventricular systolic function is traditionally assessed from M-mode or 2D measurements. The simplest form of measurement is fractional shortening (FS). This can be performed by M-mode or 2D imaging by determining the change in left ventricular internal diameter taken during systole (LVIDs) or diastole (LVIDd) during a single beat ( Fig. 29.10 ):

Normal values are 28% to 45%, with lower values indicating decreased systolic function and higher numbers indicating hyperdynamic function. FS has the advantage of being very quickly obtainable, but the measurement looks at left ventricular function across a single plane only. Thus, it is not an assessment of global function and will not detect regional wall motion abnormalities. 2D imaging can also be used to calculate the left ventricular ejection fraction (EF). In this calculation, assumptions are made about the left ventricular geometry in order to calculate diastolic and systolic volumes from 2D imaging. The EF is then determined by calculating the percentage of volume ejected during a single heart cycle derived from the diastolic volume (Vd) and systolic volume (Vs) using the following equation:
2D measurements of left ventricular EF can be obtained by Simpson’s biplane method using apical 4-chamber and apical 2-chamber views or from the area-length method using a combination of subcostal coronal or apical 4-chamber view with subcostal sagittal or parasternal short-axis view ( Fig. 29.11 ). A normal EF is 55% or above, with values of 45% to 50% indicating mild dysfunction, 35% to 45% indicating moderate dysfunction, and measures below 35% representing severe left ventricular systolic dysfunction. The left ventricular volumes are based on assumptions of a conal shape and that spinning the left ventricle along its long axis will yield an accurate estimation of left ventricular size. This assumption becomes less accurate in a dilated left ventricle in which the left ventricle is more spherical or in certain congenital heart diseases that may alter left ventricular geometry. Accuracy of the calculation will be affected by any regional wall motion as can be seen with volume or pressure load on the right ventricle affecting septal wall motion, and with ischemia or conduction abnormalities that can affect various walls.

3D imaging can also be used to calculate an EF (see Fig. 29.2 ). 3D EF allows for better detection of abnormalities in function due to regional wall motion abnormalities and correlates well with magnetic resonance imaging (MRI) findings of left ventricular function in children and adults with congenital heart disease. A limitation of left ventricular functional assessment by 3D imaging is artifact related to respiration and longer imaging times. Additionally, the image must be of high enough quality to allow for clear delineation of the endocardium along the entire left ventricle in order to permit accurate tracing of the left ventricular chamber. A large body habitus, lung interference, or bandages can make obtaining adequate images difficult. Use of ultrasonic-enhancing contrast agents can produce a clearer delineation of the endocardial border. However, these agents are contraindicated in the presence of a right-to-left shunt, which limits its use in congenital heart disease.
These measurements are all dependent on loading conditions. In the setting of high afterload, the wall motion (FS and EF) may be severely decreased despite preserved contractility. The stress-velocity index attempts to account for the loading condition by assessing wall stress, which takes into account wall thickness and pressure generation, against wall motion as measured by rate-corrected circumferential fiber shortening (Vcfc) and can help determine if an abnormality in wall motion is due to excessive afterload or decreased contractility. There is an inverse linear relationship between Vcfc and end-systolic wall stress. Although load independent, the calculation for Vcfc is based on the same measurement as FS; therefore, the calculation is accurate only for a normally shaped left ventricle.
Spectral Doppler and tissue Doppler imaging (TDI) can also be used to assess left ventricular systolic function. When an adequate jet of mitral regurgitation is present, the mean change in left ventricular systolic pressure (LV dP/dt) can be obtained from measuring the acceleration time between 1 m/s and 3 m/s. A value of 1200 mm Hg/s or greater is considered normal. This is a nongeometric measurement and has been shown to correlate with MRI-derived assessment of left ventricular systolic function in patients with Fontan physiology. LV dP/dt can be particularly useful when bandages or other causes of poor acoustic windows prohibit obtaining adequate 2D imaging for assessment of function. TDI can be used to measure peak S′ wave from lateral or septal wall as an easy measure of left ventricular systolic function that can often be obtained in patients with suboptimal images for other measures of function. TDI can also be used to calculate the myocardial performance index (MPI; Fig. 29.12 ). The MPI assesses elements of both systolic and diastolic function. The normal left ventricle MPI value in children is 0.32 ± 0.06.

Right ventricular systolic function
The complex shape of the right ventricle makes calculation of right ventricular systolic function more difficult than that of the left ventricle. Therefore, a qualitative assessment of right ventricular systolic function often is preferred. Conversely, multiple quantitative measures can be assessed and both tricuspid annular plane systolic excursion (TAPSE) and right ventricular fractional area change (FAC) are recommended for assessment of right ventricular systolic function. Perhaps the simplest measure of right ventricular systolic function is TAPSE, which is obtained by placing M-mode through the tricuspid valve from an apical view ( Fig. 29.13 ). Although validated for use in pediatrics, alterations to right ventricular geometry can affect its accuracy in measuring right ventricular function. Right ventricle FAC is similar to left ventricular EF in that it is a volumetric assessment of right ventricle function, but it does not take into account the entire right ventricle volume and has suboptimal reproducibility. Similar to the left ventricle, TDI can be used to obtain S′ wave and MPI, with a normal right ventricle MPI value of 0.28 ± 0.04. 3D assessment of the right ventricle may be the most accurate measurement of right ventricular systolic function; it has good correlation to MRI findings in children after surgical repair of congenital heart disease. However, 3D assessment of the right ventricle is time-consuming and requires special software that is not readily available.
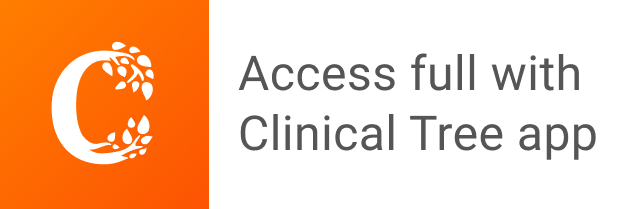