Dendritic cells (DCs) together with monocytes and macrophages compose the mononuclear phagocyte system (MPS).
DCs are professional antigen-presenting cells (APCs), abundant at body surfaces and within tissues, where they sense and sample the environment for self and non-self antigens.
Three major subsets of DCs—plasmacytoid DCs (pDCs), conventional DCs (cDCs), and monocyte derived DCs (moDCs)—are characterized by distinct origins, receptors, and functions.
Upon antigen capture, DCs undergo a process of “maturation” exemplified by enhanced antigen processing, induction of major histocompatibility complex (MHC) molecules, co-stimulatory molecules (CD80/86), and cytokine production. DCs migrate to primary and secondary lymphoid organs, where they present processed antigens to naïve T cells to induce immunity or tolerance.
Mature DCs acquire the ability to differentiate naïve T cells into T helper (Th) 1, Th2, or Th17 cells, T follicular helper (Tfh), or regulatory T cells (Treg). Maturing DCs also express cytokines that enable the activation of B cells and natural killer (NK) cells and promote the recruitment of other innate immune effector cells.
DCs are innate immune phagocytic cells, which sense the environment through membrane and cytosolic pattern recognition receptors (PRRs). DCs contribute to the maintenance of tolerance in the thymus and in the periphery, although their functions remain yet partially understood.
There is a fine equilibrium in DC regulation between tolerogenic and inflammatory states, which can contribute to autoimmune diseases or to tolerance of tumors.
DCs, as one of the main regulators of the immune response, can either be targeted or used as adjuvants to contain immunopathologies such as autoimmune diseases and cancers, respectively.
Introduction
In 1973 Ralph Steinman and Zanvil Cohn identified a new cell type in murine splenic cultures. They described these as adherent nucleated cells with multiple pseudopods. Owing to their unique morphology, they named them dendritic cells (DCs). A decade later, in 1983, Steinman and Nussenzweig demonstrated that DC depletion completely ablated the stimulatory capacity in murine mixed lymphocyte reactions. Further research revealed that DCs are specialized in acquiring and presenting antigens to T cells and have superior capacity over other antigen-presenting cells (APCs) in activating cytotoxic T lymphocytes (CTLs). , Moreover, a small number of DCs can directly or indirectly activate a large number of T cells, B cells, natural killer (NK) cells, and NK T cells, thus improving the efficiency of the immune response. Furthermore, DCs express a plethora of activating and co-stimulatory receptors and ligands. As such it is no surprise that DCs are often referred to as “nature’s adjuvants.”
Under normal circumstances DCs exist in an immature form, populating practically every tissue in the body. DCs are activated upon encountering pathogen-associated molecular patterns (PAMPs) or danger-associated molecular patterns (DAMPs), which leads to a process of “maturation” that involves changes in the DC phenotype, antigen acquisition capacity, migration, and an ability to traffic to draining lymph nodes, where they prime cellular and humoral adaptive immune responses. Upon maturation, DCs process antigens (self and non-self) and then present them to antigen-specific adaptive immune cells (T cells and B cells) to induce immune responses to contain diseases such as cancer or infections, but also to maintain tolerance to self-antigens. This chapter provides our current understanding of DC ontogeny and function as well as DCs’ potential in clinical applications as immunotherapeutic agents.
Dendritic Cell Subsets and Development
DCs have been classified into subsets based on their location, function, and origin. It is currently believed that all DC subsets undergo four stages of development, namely hematopoietic precursors, DC precursors (pre-DC), immature DCs, and mature DCs. Pre-DCs are continuously produced at a steady rate in a pathogen-independent manner from CD34 + hematopoietic stem cells (HSCs) within the bone marrow. Fms-like tyrosine kinase-3 ligand (Flt-3-L) and granulocyte-macrophage colony-stimulating factor (GM-CSF) represent key DC growth and differentiation factors. The development of stromal cell culture systems, comprising of co-cultures of cord blood isolated CD34 + HSCs and murine bone marrow stromal cells (OP9 cells) in the presence of factors such as stem cell factor (SCF), GM-CSF, and Flt3L have led to the identification of definitive pre-DCs that give rise to DC subsets found in the blood. In humans, all DCs originate from granulocyte monocyte dendritic cell precursors (GMDP), which develop into monocyte dendritic cell precursors (MDP) within the bone marrow. MDPs subsequently give rise to two distinct lineages, the common dendritic cell progenitor (CDP) and the common myeloid progenitor (CMP). The CDPs differentiate into pre-DCs that migrate out of the bone marrow and give rise to two classical DC subsets, the conventional DCs (cDCs) and plasmacytoid DCs (pDCs), whereas the CMPs differentiate into inflammatory DCs (iDCs), found in blood ( Fig. 9.1 ).

For ex vivo studies and phenotyping in humans, DCs are identified as cells lacking the expression of lineage markers CD3 (T cell marker), CD19, and CD20 (B cell markers), CD14 (monocytic marker), and CD56 (NK cell marker) and as being positive for HLA-DR. Terminally differentiated DCs are broadly classified into four subsets, namely cDCs, pDCs, iDCs, and Langerhans cells (LCs). However, recent fate mapping studies have contested the classification of LCs as DCs, arguing that LCs fall into the tissue macrophage category.
Conventional Dendritic Cells
cDCs comprise two subsets in humans and mice, cDC1 and cDC2 (see Fig. 9.1 ). In humans, cDC1s are commonly phenotyped by surface expression of Clec9a, CD141, low CD11c, XCR1, and CADM1 ( Fig. 9.2 ) while murine cDC1s express CD8a and/or CD103. The cDC2 cells in humans are typically CD1c + , and they express high CD11c, CD11b, and CD172a (see Fig. 9.2 ). In mice they are CD4 + and CD11b + . While both cDC1 and cDC2 can activate CD4 + and CD8 + T cells, several key differences distinguish cDC1s from cDC2s. For instance, cDC1s are considered more adept at antigen cross-presentation and at CD8 + T cell activation than any other APCs, including cDC2s. Cross-presentation is a unique antigen-presentation pathway that allows APCs to present exogenous antigens on class I MHC molecules; this pathway is described in detail later. Second, although cDC1 can be found in blood in small numbers, these cells are thought to primarily reside in the lymph node, while the cDC2s are migratory cells, outnumbering cDC1s in peripheral tissues by several fold.

A key difference between DCs is the array of pattern recognition receptors (PRRs) expressed by each subset. PRRs are an important class of receptors that recognize pathogen-associated molecular patterns (PAMPs, expressed by microorganisms) or danger-associated molecular patterns (DAMPs, secreted by damaged cells). PRRs include secreted receptors such as MBL, CRP, SAP, LBP; cell-surface receptors like Toll-like receptors (TLRs1/2/4/5/6), CD14, MMR, MSR, MARCO, and intra-cellular receptors such as TLRs3/7/8/9, RIG-I and MDA5, STING, DAI, AIM2, and NOD receptors. cDC1s primarily express TLR3 and TLR8 and in response to their stimulation secrete IL-12 as well as Type-III interferons. By contrast, cDC2s express and respond to a wider range of TLRs.
The cDC1 and cDC2 cells exert distinct transcriptional programs. cDC1s express interferon response element-8 (IRF8), Batf3, Bcl16, and Flt3, whereas the cDC2s express IRF4, Csl, and Klf4. , Notch signaling is a unique cell-to-cell signaling pathway that controls development of several immune cells including DCs. Stimulation of transmembrane Notch receptors by Delta-like ligands (DLL) 1, 3, and 4, or Jagged ligands (Jagged 1 and 2), leads to gamma-secretase driven Notch receptor cleavage. This event is followed by nuclear translocation of Notch intra-cellular domain and interaction with the transcription factor Csl (or RBPJ in mice). Overall, this pathway facilitates cellular development, maintenance, and differentiation programs. The role of Notch signaling in DC differentiation has remained controversial with different research models yielding conflicting reports. However, two independent groups recently confirmed the importance of Notch signaling in DC programming. Using the OP9 feeder culture system, Notch-2 signaling regulated pDC versus cDC differentiation from the precursor cells in both mice and humans. , Specifically, in the context of human cells, investigators demonstrated that expanding CD34 + cells (from either human cord blood or peripheral blood mononuclear cells [PBMCs]) with Flt3L, S-CSF, IL7, and thrombopoietin (TPO) for 7 days followed by co-culture with OP9 cells yielded a high number of pDCs but not many cDC1s. However, co-culturing CD34 + cells with a mixture of OP9 and OP9_DLL1 cells (OP9 cells expressing Notch ligand DLL1) remarkably increased the yield of cDC1s while moderately inhibiting the yield of pDCs. These Notch-dependent cDC1 cells were functionally and transcriptionally similar to cDC1s found in blood, suggesting the significance of Notch signaling for cDC1 differentiation in vivo. Other studies in murine models have emphasized the role of Notch signaling in cDC2 development, particularly in context of T follicular cell activation and induction of B cell response during infection.
Plasmacytoid Dendritic Cells
pDCs are distinguished by expression of CD123, CD303, CD304, and lack of CD11c (see Fig. 9.2 ). The geospatial distribution of pDCs is more restricted than cDCs. Although small numbers of pDCs can been found in blood and tissues such as nasal mucosa, pDCs primarily populate T cell areas of lymphoid tissues. The production of extraordinarily high levels of Type-1 interferon is unique to this cell type and is important for initiating a strong antiviral innate response, promoting maturation of bystander CD11c + cDCs and protecting cDCs from the cytopathic effect of viruses. This response is primarily enabled by high expression of TLR7 and TLR9 on pDCs and their ability to rapidly present intra-cellular antigens, like those derived from viruses, to CD8 + T cells. ,
Interestingly, upon activation, pDCs have been reported to differentiate into cells bearing similar characteristics to activated cDCs (such as high expression of class II MHC molecules and the capacity to prime naïve T cells) but express low levels of CD11c and lack the typical myeloid markers. , All these studies highlight the complexity and plasticity of DC development.
Inflammatory Dendritic Cells
iDCs arise from monocytic precursors during infection and inflammation in blood and migrate to the site of inflammation. iDCs are characterized by surface expression of CD1c, high expression of CD11c, CD11b, CD172a, and CD14 (see Fig. 9.2 ) and MCSFR and ZBTB46 as transcription factors. iDCs can process and present antigens to both CD4 + and CD8 + T cells; however, their exact functional profile in vivo remains undetermined. It is postulated that the monocyte-derived dendritic cells (moDCs) ( Fig. 9.3 ), used in most DC-based cell therapy vaccines, closely resemble the in vivo iDCs. moDCs are generated from monocytes isolated from patient blood, and ex vivo differentiated into immature DCs under the influence of GM-CSF and IL4.

Langerhans Cells
LCs are self-renewing epidermal myeloid cells located in the epidermis, where they form a network between keratinocytes. LCs are also observed in squamous stratified epithelia, such as bronchiolar tissue, and in oral and genital mucous membranes. They can migrate and mature into DCs. LCs express shared common markers, such as CD11c, CD1a and the C-type lectin receptor (CLR) langerin (CD207) (see Fig. 9.2 ). A skin homing receptor, cutaneous lymphocyte-associated antigen (CLA), has been implicated in regulating the migratory potential of LCs. , Langerin, a common marker of LCs, delivers antigen through receptor-mediated endocytosis to Birbeck granules; these granules are structures connected to the endosomal network that are implicated in canonical and non-canonical antigen processing and presentation. LCs were initially classified as DCs due to their function as APCs, their capacity for migrating to lymph nodes, and inducing T cell activation. However, recent developments in fate mapping studies suggest that LCs originate from the yolk sac progenitors and the fetal liver and differentiate into specialized tissue resident macrophages. ,
New Developments in Dendritic Cell Classification
Using single-cell RNA sequencing, a recent study analyzed blood DCs with unbiased gene clustering and identified six DC subsets (DC1-DC6) . The new classification emerging from this study consists of (see Fig. 9.2 ):
- •
The DC1 and DC6 subsets corresponding to predefined cDC1 and pDC subsets.
- •
The DC2 (CD1c+_A) and DC3 (CD1c+_B) subsets were identified as two unique clusters under the cDC2 subset. Both DC2 and DC3 subsets express CD1c, CD11b, and CD11c but unlike DC2 , the DC3 subset was enriched for CD14 and acute and chronic inflammatory gene set.
- •
The DC4 subset, negative for both CD141 and CD1c, was found enriched for CD16 and in genes involved in Type-I interferon secretion and response to virus.
- •
A novel but small subset, DC5, did not cluster with any other blood DC subset and can be identified by the expression of Axl and Siglec6, earning the name “AS-DCs.” Despite sharing many markers with pDCs, AS-DCs are functionally, phenotypically, and morphologically distinct from pDCs.
These results were corroborated by investigators, who employed flight mass cytometry (CyToF) to identify DC subsets in blood and tissue. The authors also reported a conserved phenotype of cDC1 and cDC2 between blood and lymphoid organs while the skin DCs displayed tissue-specific phenotype with considerable inter-individual heterogeneity. However, the precise functionality of these newly discovered subsets remains to be determined.
In addition to subsets mentioned previously, other groups, over the years, have described atypical DC subsets that do not identify with any classical DC subset. These include a small pool of migratory precursor cells that originate from the CDPs in the bone marrow and can only differentiate into either CD1c + or CD141 + cDCs. A group of CD103 + DCs in the intestinal LNs has been reported to induce expression of gut homing receptor, CCR9 on T cell and B cells through a retinoic acid receptor-dependent mechanism. Furthermore, intestinal CD103 DCs process dietary vitamin A into retinoic acid to support the differentiation of gut-homing FoxP3 + regulatory T cells (Tregs). ,
Dendritic Cell Maturation and Activation
DC maturation is a complex process that relies on key functional properties, such as activation by environmental sensing, antigen uptake, processing and presentation, migration, and T cell stimulation. The DC process of maturation is related to their environment and is therefore heterogeneous. It confers specific and distinctive functional properties to different DC subsets.
DC maturation can be prompted by several activating cues such as pathogen or nonpathogen derived factors, cellular debris, stress signals, and receptor-ligand interaction, among others. In the steady state, cDCs are in a quiescent state that is characterized by a low surface expression of MHC molecules and co-stimulatory molecules, such as CD80, CD83, CD86, and CD40. Immunogenic conditioning of cDCs induces expression of class I and II MHC, T cell co-stimulatory molecules, such as CD80 and CD86, cytokines (e.g., TNF, IL-12, IL-18), and chemokines (e.g., RANTES, MIP-1α, IP-10). Additional changes include induction of CCR7, CCL19 and CCL21-dependent migration to follicular T cell–enriched zones in lymphoid organs and downregulation of receptors that serve to retain DCs at the site of activation (such as receptors for CCL3, CCL4 and CCL5). Upon antigen uptake the DCs are programmed to dampen antigen acquisition and processing and promote antigen presentation. DCs acquire the ability to induce clonal expansion and differentiation of antigen-specific naïve T cells into effector T cells.
The activation and maturation event also polarizes the DCs toward a tolerogenic or immunogenic phenotype. Based on their environmental conditioning, DCs regulate the adaptation of T cell polarization to the specific nature of the stimuli. For example, activation of DCs in the absence of help from CD4 + T cells, activation with suboptimal or prolonged innate stimuli, or maturation in response to stimuli like thymic stromal lymphopoietin (TSLP) which leads to induction of co-stimulatory molecules but does not induce an inflammatory cytokine response, can all lead to tolerogenic DCs. In the steady state, tolerogenic DCs are thought to present self-antigen and promote peripheral tolerance. However, in events of tumorigenesis or infection, tolerogenic DCs promote tumor evasion and inhibit pathogen clearance, respectively. Indeed, tolerogenic DCs have often been observed in cases of chronic activation such as in cancer. Moreover, several pathogens have developed specific mechanisms of immune evasion via induction of tolerogenic DCs.
Activation allows immature DCs to acquire a diverse array of antigens from pathogens, exosomes, apoptotic cells, etc. Pathways of antigen acquisition include, micropinocytosis, macropinocytosis, receptor-mediated endocytosis, and phagocytosis. Activation and maturation via pattern recognition receptors (PRRs) is a key initial step in antigen acquisition, processing and presentation.
Pattern-Recognition Receptors and Dendritic Cell Maturation
DCs sense their environment through a broad panel of PRRs. PRRs are highly conserved innate immune receptors that detect PAMPs or endogenous DAMPs. PRRs are extremely diverse, detecting a wide range of molecular patterns, including proteins, lipids, carbohydrates, nucleic acids, and mineral crystals. PRRs include the Toll Like Receptors (TLRs), the C-type lectin Receptors (CLRs), the Nod-like receptors (NLRs), the retinoic acid–inducible gene (RIG)-I–like receptors (RLRs), the scavenger receptors (SRs), the integrins, and others such as the Ig Fc receptors (FcRs). Triggering of PRRs such as TLRs or C-type lectins on DCs is thought to be critical for their functional maturation and the priming of T cell responses to infection, therefore coupling innate and adaptive immunity.
RLRs and Intra-cellular DNA Sensors
RIG-I and MDA5 RNA helicases are intra-cellularly expressed PRRs involved in the recognition of intra-cellular RNA upon viral infection while cGAS and AIM2 receptors recognize intra-cellular DNA.
TLRs
TLRs are a prominent class of PRRs that respond to PAMPs, such as peptidoglycan, lipopolysaccharide (LPS), and flagellin, and regulate DC functions. TLRs can be grouped into two subfamilies based on their subcellular localization: the TLRs expressed on the cell surface (in humans and mice, TLR1, TLR2, TLR5, and TLR6, and restricted to mice, TLR11, TLR12, and TLR13) and those localized to specialized endosomal compartments (TLR3, TLR7, TLR8, and TLR9). Finally, TLR4 can localize to both sites. Each DC subset expresses distinct panels of TLRs, which modulate their functional specialization. Human pDCs express high levels of nucleic acid-specific TLRs such as TLR7 and TLR9. TLR7 and TLR9 detect ssRNA and unmethylated cytosine-phosphatidyl-guanine (CpG) DNA, respectively, which are motifs usually found in both bacteria and viruses. TLR8 also recognizes bacterial ssRNA. Human CD1c + cDCs express mostly TLR1 through TLR8 and TLR10, whereas the CD141 + cDCs express TLR3 and TLR7/8 and low levels of TLR1 and TLR2. Activation of DCs in response to binding of TLR agonists is controlled by signaling through the Toll/IL-1 receptor (TIR) domain present in the cytoplasmic domain of TLRs. TLR stimulation by self- and non-self-antigens can regulate cytokines such as TNF, IL-12, IL-6, IL-23, and IL-17, which have an important role in the polarization of T helper cell subsets during priming by DCs, and thus the functional consequences of DC activation.
NLRs
Another important inflammatory signaling circuit governed by PRRs is the inflammasome pathway. The inflammasome is a multiprotein complex that can be activated through NLRs like NLRP3, NLRC4, or NLRP1 through PAMPs (such as pore-forming toxins, RNA and DNA, flagellin, β-glucans, and zymosan) or DAMPs (such as adenosine triphosphate [ATP], uric acid crystals, amyloid β, alum, silica and asbestos). Inflammasome activation induces the activation of key inflammatory cytokines, such as IL-1β and IL-18, and regulates an inflammatory cell death pathway known as pyroptosis. Each NLR regulates a unique inflammasome activation cascade. NLRP3 inflammasome is activated in a two-step process. The first step requires TLR2 or 4 driven upregulation of key inflammasome components, namely NLRP3, ASC, pro-IL-1β, and pro-IL18. The second step involves NLRP3 activation, assembly of the inflammasome complex, caspase1 cleavage and subsequent release of cytokines and possibly the induction of pyroptosis.
CLRs
C-Type lectins are another family of PRRs that recognize sugar motifs in a calcium-dependent manner through their carbohydrate recognition functional domain. The repertoire of CLR expression varies among the different DC subsets and is tightly regulated by the interactions with the environment. CLRs are involved in endocytosis, phagocytosis, antigen processing, and presentation through regular class II MHC or by class I MHC cross-presentation. Several CLRs, such as DEC205, mannose receptor (MR), and DC immunoreceptor (DCIR), contribute to the cross-presentation of endocytosed antigens. DC-SIGN, a CLR expressed on many DCs is well known for interacting with pathogens like HIV-1 and 2, SIV etc. Some CLRs are mostly restricted to specific DC subsets such as langerin on LCs. BDCA-2 expressed by pDCs shares similar immunomodulatory properties as DEC-205 and has been targeted by recombinant antigens bound to antibodies in order to modulate DC function. This is accomplished by inducing antigen-specific tolerance or immune stimulation with the addition of a maturation signal. Finally, many CLRs recognize ligands expressed by apoptotic or necrotic cells and participate in the uptake of these dead cells by DCs, although most of the clearing process is mediated by neutrophils and macrophages. DNRG1 (CLEC9a) is expressed on CD141 + DCs and recognizes F-actin, which is expressed at the surface of necrotic cells. This interaction induces immunogenic responses and promotes the cross-presentation of processed necrotic antigens on class I MHC. In the same way, macrophage-inducible C-type lectin (MINCLE), which is another CLR that recognizes necrotic cells, can induce inflammatory responses.
Fc Receptors
FcRs, expressed by many immune cells, are important in regulation of immune responses to immune complexes composed of antigen bound to antibody and sometimes to components of the complement system. DCs express activating receptors FcγRI, FcγRIIA, FcγRIIIA, and inhibitory receptor FcγRIIB. Infected cells or pathogens coated with IgG activate FcγR-mediated clearance by antibody-dependent cell-mediated cytotoxicity (ADCC) or phagocytosis and/or indirectly through the release of cytokines.
In addition to the receptors mentioned above, scavenger receptors (SRs) like CD36, CD-205, LOX-1, to name a few, promote DC activation and maturation. These receptors also regulate multiple cellular functions like pathogen clearance and apoptotic cell clearance. For example, CD36 on DCs detects phospholipid phoshatidylserine on apoptotic cells and facilitates apoptotic cell clearance. LOX-1 promotes DC activation by oxidized low density lipoprotein (LDL) and contributes to artheroscelorosis. Complement receptors on DCs regulate opsonization of pathogens and dying cells. DCs also express receptors that specifically bind pathogens like CD4, CCR5 and CXCR4 that bind HIV. Other receptors aid in activating atypical immune cells. For example, the CD1 family of receptors allow DCs to present antigens such as sphingolipids, sulfatides and glycosphingolipids to activate γδT cells, and NK T cells. Hence, many distinct families of receptors, adorn and regulate DC function. , ,
Over the past decade it has become evident that DC activation, especially through TLRs, significantly influences not just antigen acquisition, but also the process of antigen presentation. TLR-induced DC activation induces an innate immune response conducive to micropinocytic antigen uptake, but only transiently, followed by a near cessation of antigen uptake.
Antigen Processing and Presentation
DCs are specialized in antigen processing and can efficiently present endogenous and exogenous antigens in both class I and II MHC contexts. This activity is influenced by the maturation and activation status of the DC, which promotes phagolysosome maturation, peptide processing, peptide macropinocytosis, and DC metabolism to promote antigen processing and presentation of antigens by class I MHC and class II MHC, as well as lipid presentation by CD1 molecules.
Major Histocompatibility Complex Class I Antigen Presentation
DCs express class I MHC molecules on their cell surface bearing self or non-self peptides derived from the cytosol. These peptides are predominantly generated from ubiquitinated nascent, misfolded, and neosynthesized defective self-proteins, or defective ribosomal products (DRiPs). In the cytoplasm, the cytosolic proteasome, comprising of interferon regulated PA28 proteasome activator and leucine aminopeptidase, cleaves these proteins into peptides of appropriate length for presentation on class I MHC molecules. , Interestingly, DC maturation enhances antigen processing through upregulation of such immuneproteosomes. After being processed in the cytoplasm the trimmed peptides are translocated to the endoplasmic reticulum (ER) through the transporter associated with antigen processing (TAP) and further shortened to 8-9mer peptides, optimal length for loading onto class I MHC ( Fig. 9.4 ). This shortening of peptides in the ER is performed by ER aminopeptidase-1 (ERAP-1). Once a stable class I MHC-antigen complex is formed, it is routed to the cell surface.

Cross-Presentation
DCs have the unique capacity to acquire antigens exogenously, internalize them, and process them for presentation on class I MHC molecules. This property is atypical because, in most cells, class I MHC molecules exclusively present endogenous proteins. Although the precise mechanism of cross-presentation remains controversial, it is well established that DCs use this process to activate CD8 + T cells. DCs acquire antigens by endocytosis in the form of apoptotic cells, necrotic cells, antibody-opsonized cells, immune complexes, heat shock proteins, and exosomes, and even by nibbling of live cells. , Two main intra-cellular pathways, the cytosolic and the vacuolar pathways, traffic exogenous antigens from phagolysosomal compartments to the ER for cross-presentation (see Fig. 9.4 ).
In the cytosolic pathway (also referred as endosome-to-cytosol pathway ), antigens are transferred to the cytoplasm from the endosomal compartment and processed by the cytosolic proteasome. Thereafter the peptides are transported back into the endosome or to the ER where they are loaded onto class I MHC molecules. Class I MHC molecules, however, need to be present in the ER or the endosomes to be available for antigen loading. For this, either newly assembled class I MHC molecules are transported into the ER or cell surface expressed class I MHC complexes are recycled back into the endosome for reloading (see Fig. 9.4 ). This pathway is sensitive to proteasome inhibitors, suggesting that antigenic-proteins access the cytosol and are degraded by proteasomes. Yet, whether the peptide loading occurs via the classical class I MHC pathway described previously or in endocytic compartments remains to be fully determined.
Antigen processing and loading through the vacuolar pathway is believed to occur in the endocytic compartments. This conclusion stems from the observations that inhibition of lysosomal proteolysis inhibits antigen presentation via this pathway, whereas inhibition of cytosolic proteasomes has no effect (see Fig. 9.4 ). While some evidence suggests that cytosolic pathway maybe the predominant antigen processing mechanism here, there is no clear indication as to the relative contribution of cytosolic pathway over vacuolar pathway. Data from several groups indicate that DCs in general and specific DC subsets such as the CD8α + DCs in mice and DC1s in humans are more specialized for cross-presentation than other APCs. , Recently, progress has been made in better understanding the cross-talk between the different cellular compartments that regulate the cross-presentation. Nair-Gupta and collaborators have identified an important role in DCs for communication between the endosomal recycling compartment (ERC), which is a key reservoir of class I MHC molecules, and the phagosome in cross-presentation. Trafficking from the ERC to phagosomes is controlled by the TLR-MyD88-IKK2 pathway, which stabilizes membrane interactions between the phagosome and the ERC, ensuring that ERC-deployed class I MHC are specifically routed to phagosomes engaged in TLR signaling. On the other hand, the class I MHC peptide loading complex (PLC) is recruited from another subcellular compartment, the ER and Golgi intermediate compartment (ERGIC), in a TLR-independent manner. The highly coordinated trafficking from ERC and ERGIC delivers essential components for cross-presentation to phagosomes during infection, and control of the ERC pathway by TLR signals favors phagosomes that contain microbial proteins for cross-presentation in the context of TLR-primed T cell co-stimulation.
Interestingly, DCs have been documented to acquire complete functional class I MHC-antigen complex from a donor cell and decorate on their own (recipient) cell surface. This mode of antigen presentation has been coined “cross-dressing” and is postulated to boost a memory like response in previously primed CD8 + T cells.
Major Histocompatibility Class II Antigen Presentation
While all nucleated cells express class I MHC, only APCs express class II MHC. Class II MHC presents short peptides to activate CD4 + T cells. Class II MHCα/β heterodimers rely on a specialized type II transmembrane chaperone protein, the invariant chain (Ii), for stable assembly in the ER. Assembled class II MHC molecules are transported and concentrated in multivesicular and multilamellar late endosomal compartments called class II MHC–containing compartments (MIICs). Antigens for class II MHC loading are acquired by APCs through macropinocytosis, receptor-mediated endocytosis, phagocytosis, and autophagy. Antigens are retained within late endosome or phagosomes before fusing with lysosomes to form phagolysosomes. Concomitant TLR signals induce activation of the vacuolar proton pump that enhances lysosomal acidification and antigen proteolysis into antigenic peptides within phagolysosomes. Acidification of this compartment allows optimal activity of cathepsin S. Cathepsin S degrades the cytoplasmic tail of Ii, leaving a short peptide, the class II MHC–associated invariant-chain peptide (CLIP), bound to the peptide-binding groove and thus protected from proteases. CLIP is replaced by an antigenic peptide through the action of the catalyst-chaperone protein HLA-DM, which accelerates the rate of CLIP release, and the loaded class II MHC molecules are thought to be transported through cytoskeletal tubular structures that are directed toward the site of T cell interaction at the plasma membrane.
DCs display several specializations in antigen processing/presentation mechanisms that distinguish them from other APCs. For instance, as opposed to macrophages that rapidly degrade internalized antigens, DCs process antigens slowly, thus allowing sufficient time for the DCs to mature and migrate to the lymphoid organs. Once mature, DCs increase proteolysis and quickly process antigens for MHC presentation for maximal T cell activation. TLR engagement regulates phagosome maturation, enhances lysosomal acidification, and increases antigen uptake transiently. Class II MHC surface expression and turnover rates are regulated by cytoplasmic domain ubiquitination in DCs. This mechanism explains the expression of low levels of class II MHC molecules by immature DCs and their increase in half-life after maturation, sustaining antigen presentation after migration into secondary lymphoid organs.
T Cell Activation
T cells are primed and activated by DCs in the lymph nodes (see also Chapter 12 ). Lymph nodes are specialized immune organs situated at the confluence of lymphatic vessels and organized into the outermost cortex and inner medulla. The medulla contains macrophages and medullary cords, and plasma cells that secrete antibodies. The cortex is organized into two sections, the para-cortex region where T cells and APCs converge, and the outer-cortex where B cells form lymphoid follicles. The T cell-rich para-cortex is also referred to as the T cell zone . Upon activation, immature cDCs migrate through afferent lymph from nonlymphoid tissues to the T cell zone in the lymph nodes. pDCs and naïve T cells migrate into T cell areas through the high endothelial venules (HEVs) of lymph nodes and marginal zone of the spleen, likely using CCR7 and CD62-L. Both activated blood cDCs and pDCs migrate in response to lymph node homing chemokines (CCL19 and CCL21) through expression of CCR7.
An inflammatory gradient guides the movement of T cells and DCs around the lymph node. However, the exact composition of this gradient is uncertain. Similarly, while DC-T cell interaction is known to take place within the para-cortex, its exact location and kinetics are unknown. Furthermore, factors such as antigen burden (replicating infectious agent vs. non-replicative vaccine antigen) and the type of antigen (blood borne, cell free lymph borne, or carried by DCs) dictate the strength and kinetics of DC-T cell interaction. Elegant intra-vital fluorescent microscopy in sophisticated murine models indicates that the lymph node-resident DCs and migratory DCs populate discrete sections of the para-cortex. The murine models demonstrate that the resident cross-presenting cDCs (most likely equivalent to human cDC1 cells, though not yet validated) accumulate in the deep-cortex in close proximity to the incoming CD8 + T cells while the migratory cDCs remain in the peripheral cortex in proximity to the CD4 + T cells. , Thus, upon encountering antigens in the tissues or blood, migratory DCs mature, process, and present these antigens on MHCs and travel to the peripheral cortex of the lymph nodes where they activate the helper CD4 + T cells. Activated CD4 + T cells in turn facilitate activation of CD8 + T cells in deep-cortex by licensing the lymph node resident cross-presenting DCs.
It is postulated that antigen-loaded DCs activate T cells over several hours and three dynamic phases requiring three distinct cell-to-cell signals. First, incoming naïve T cells survey DCs in short bursts to find a matching antigen-MHC combination, aggregating each antigen specific signal. Second, once the activation signals reach a cumulative threshold, T cells initiate a sustained engagement with DCs, leading to their activation. Induction of memory T cells also takes place during this step. In the third phase, T cells start proliferating and break the prolonged DC engagement, reverting to transient contact. Cell-free antigens that enter the lymph node though passive diffusion in the lymph are processed by a specialized DC subset residing within the lymphatic sinus epithelium. These DCs can rapidly activate T cells, bypassing the lengthy three phase process mentioned previously. After clonal expansion, the activated antigen specific T cells exit the lymph node and go back in the circulation to locate their target cells.
During T cell activation, several factors influence generation of CD8 + memory T cells, such as the load of antigen on DCs and frequency of CD8 + T cell precursors. Moreover, generation of effective CD8 memory T cells requires CD4 + T cell help in the form of secreted IL-2 and CD40L-CD40 interaction. However, if the frequency of antigen specific CD8 + T cells is sufficiently high, the need for CD4 + T cell help may be dispensable for CD8 + T cell activation, but might still be required to establish memory responses, to protect primed CD8 + T cells from receptor mediated cell death and to avoid T cell exhaustion.
Perhaps due to the army of co-stimulatory molecules involved in DC-T cell synapse, a small number of MHC-peptide complexes (<200) on the surface of mature DCs are sufficient to elicit a large T cell response, making DCs up to 1000 times more efficient at T cell activation than other APCs. The strength and duration of immunological synapse between T cells and DCs dictates the quality of T cell response based on three signals. Signal 1 is generated when the T cell receptor (TCR) engages a peptide–MHC complex on the APC. Signal 2 is generated when co-stimulatory molecules on APCs engage their ligands on T cells. This signal determines the qualitative and quantitative elements of T cell activation and differentiation, and is required for priming of naïve T cells. Co-stimulatory molecules include the CD80 and CD86 members of the B7 family, which ligate to CD28 on T cells, and members of the TNF family, such as CD40. Other molecules play inhibitory roles upon encountering their receptors on T cells. For example, ICOS-L is present on both DC and B cells and engages ICOS on T cells to modulate T cell activity. Programmed death ligand 1 (PDL1) on DCs interacts with PD1 on T cells to downregulate T cell responses. It should be noted that many inhibitory TCRs like ICOS and PD1 are required to initiate T cell activation and in early stages of activation serve as markers of effector T cell functions. For example, mice lacking the expression of ICOS or ICOS-L failed to mount anti-tumor responses upon receiving anti-CTLA4 checkpoint therapy. Moreover, emerging data indicate that CD80 on DCs interacts in cis with PDL1. Such CD80-PDL1 interaction on the DC cell surface inhibits binding of PDL1 with PD1 expressed on T cells, thus preventing T cell exhaustion. Finally, signal 3 is derived from cytokines secreted by APCs. Cytokines such as IL-12 (for Th1) or IL-4 (for Th2) determine the skewing of the T cell response such that T cells may terminally differentiate toward either IFN-gamma producing Th1 cells (under the influence of IL-12), which eradicate intra-cellular pathogens (bacteria or viruses), or into IL-4, IL-5, and IL-13 producing Th2 cells (under the influence of IL-4), which promote elimination of extra-cellular infections. In addition, the signal 3 can also prompt differentiation into IL-10-secreting Treg cells that dampen Th1 response.
Priming of naïve T cells by DCs into Th1 or Th2 cells is determined by multiple factors. DCs require expression of the transcription factor T bet for Th1 priming. Activation of DCs with immunosuppressive stimuli like TSLP induces Th2 priming. Prolonged and low level of DC activation exhausts the DCs inducingTh2 priming. Low and high antigen dose induces Th2 and Th1 priming, respectively.
In the past years, new CD4 + T cell lineages have been discovered. These include Th9, Th22, and Th17. Of these, the Th17 lineage is best characterized. Pro-inflammatory Th17 cells expressing IL-17 and the RAR-related orphan receptor γ (RORγt) transcription factor are aberrantly activated in multiple inflammatory disorders. Upon TLR stimulation, innate immune cells, including DCs, secrete IL-6, IL-23 and IL-1β. These cytokines, aided by TGF-β (secreted by other cells e.g., tumor cells) induce Th17 differentiation, subsequent production of IL-21, and expression of IL-23R. IL-21 amplifies Th17 differentiation and IL-23, which is produced by DCs, is needed for the maintenance of Th17 cells. IL-1β and TGF-β are critical amplifiers of Th17 differentiation, especially in humans. IL-1β and IL-6 also promote the reprogramming of forkhead box P3 + (FoxP3 + ) Tregs to Th17. Hence, the pathological effect of Th17 is highly dependent upon the availability of other cytokines in the vicinity that could overtly amplify Th17-driven inflammation.
Moreover, the signals received from priming DCs also impact the pathogenic potential of the T cells. Indeed, priming by immature DCs skews differentiation towards immunosuppressive Tregs. , However, even mature DCs have been reported to skew T cell differentiation to Tregs.
Evidence is accumulating that pDCs, which were believed to play a role only in the innate immune response because of their ability to produce high levels of IFN-I, can present viral and tumor antigens to initiate both CD4 + and CD8 + T cell responses. pDCs mature in response to viral infections (e.g., influenza and HIV), thereby providing an important link between innate and adaptive arms of the immune response. Studies using genetic depletion of pDCs in mice indicate that pDCs are primarily required to boost weak antiviral cytotoxic CD8+ T cell responses and may be dispensable against viral infections that elicit a robust CD8+ T cell response.
B Cell Activation
B cells express both antigen-specific B cell receptors (BCRs) and various TLRs, thus allowing them to play a role in innate and adaptive immunity (see also Chapter 13 ). DCs present processed antigens to naïve T cells, while B cells recognize antigen in its unprocessed native state. DCs can activate B cells in an antigen-specific CD4 + T cell–dependent manner. This action results in B cell activation and isotype switching to IgG, IgA, and IgE, as well as memory B cell generation in response to T-dependent antigens. DCs can also stimulate B cell proliferation by expression of B cell–activating factor (BAFF) and its closely related tumor necrosis family member APRIL (a proliferation-inducing ligand). Furthermore, inflammatory cytokines secreted by DCs can also affect B cell activation. IFN-α and IL-6, or ICAM-1, expressed by activated pDCs, regulate B cells to differentiate into plasma cells for T cell–independent antibody production. IFN-α can enhance antibody secretion in vivo and can induce cDCs to produce BAFF and APRIL, which trigger isotype switching independently of CD40 ligation. , In addition follicular DCs support B cell memory by continuously stimulating the B cells with antigen-antibody complexes in the germinal centers of the lymph nodes.
Cross Talk Between Dendritic Cells and Innate Lymphoid Cells
Innate lymphoid cells (ILCs) are a recently described family of lymphoid cells that lack the ability to recognize antigens via rearranged receptors such as those expressed by T cells and B cells. ILCs can be classified into three different groups based on their immune function, the transcription factors they express, and the cytokines they produce upon activation. ILCs are either cytotoxic, like the well-described NK cells, or “helper” ILCs, such as ILC1s, ILC2s, and ILC3s. ILC1s express T-bet and produce IFNγ to promote macrophage activation. The closely related ILC3s express RORγt, produce IL-22, and play a role in maintaining intestinal homeostasis. ILC2s are defined by their expression of IL-4, IL-5, IL-13 and their role during extra-cellular parasite infection and against allergens. The interactions between DCs and ILCs are complex and further define the importance of DCs as a critical link between innate and adaptive immunity.
DCs can promote ILC3 differentiation into ILC1s through IL-12 production. However, this differentiation is reversible, and is notably controlled by the presence of IL-23, IL-1β, and retinoic acid produced by DCs in the inflamed gut. DCs’ ability to produce retinoic acid directly improves ILC3 activity by upregulating RORγt and IL-22 expression. , Finally, retinoic acid also controls ILCs tissue localization, by promoting a “switch” in ILCs expression of homing receptors, from lymphoid to gut.
ILCs can also regulate DCs. ILC2-expression of IL-13 controlled the migration of activated lung DCs into draining lymph nodes, leading to the priming of naïve T cells and their differentiation into Th2 cells. In the pancreas, IL-33-stimulated ILC2s produce IL-13 and GM-CSF, leading to retinoic acid production by DCs. Finally, ILC3s activate DCs through the expression of membrane-bound lymphotoxin, , which allows them to modulate adaptive immune response through control of DC functions. It is interesting to observe that ILCs can promote T cell activation through DCs, and that DCs can in turn improve ILCs functions, providing theoretical basis for the establishment of positive feedback loops between ILCs, T cells, and DCs.
The interactions between NK cells and DCs are better characterized. Direct interactions between NK cells and mature DCs can result in NK cell activation as well as the potentiation of their cytolytic activity, and conversely, NK cells can induce further DC maturation. NK cells and DCs can form an immune synapse, probably helping directional and confined secretion of cytokines as well as facilitating receptor–ligand interactions. Activated NK cells induce DC activation through both cell-to-cell contact (involving NKp30) and TNF and IFN-γ secretion. In turn, activated DCs secrete IL-12/IL-18, IL-15, and IFN-α/β, which enhance IFN-γ secretion, proliferation, and cytotoxicity of NK cells. In some conditions, NK cells can lyse DCs through NKp30, although mature DCs are protected from cytolysis. This might represent a form of “cellular editing” whereby immature and tolerogenic DCs are cleared by NK cells in the course of an ongoing immune response.
It is thus possible that DCs and NK cells play complementary roles in sensing pathogens, such that DCs could be the first to detect microbes through their expression of PRR (TLR, NOD proteins), whereas NK cells may get activated in the absence of overt inflammation but in the presence of ligands for activating NK-cell receptors, for example in tumors. Tumor cells frequently lose class I MHC expression or express NKG2D ligands, such as MIC-A/B. In both situations, either DCs or NK cells could create an inflammatory environment and induce the integrated activation of other cell types. Thus, in mice, infection by murine cytomegalovirus (CMV) induces pDCs to secrete high levels of IFN-α/β, but CD8α + DC are the major producers of IL-12, and resistance to the virus is associated with expansion of Ly49H + NK cells, driven by IL-12/IL-18. The interaction between NK cells and DCs likely takes place early during the course of an immune response. This allows DC to exploit the ability of NK cells to kill tumor- or virus- or parasite-infected cells and to cross-present this material to T cells.
Activation of Other Elements of the Immune System
Apart from interactions with B cells, T cells and ILCs, DCs also regulate many other aspects of the immune system. For example, DCs boost anti-tumor responses by processing and presenting the synthetic ligand α-galactosyl ceramide on CD1 molecules to activate NK T cells. Similarly, DCs engage CD1-restricted γδT cells to induce inflammatory response against pathogens such as, Mycobacterium tuberculosis . This interaction matures the DCs prompting secretion of IL-12, and induction of IFN-γ secretion by activated γδT cells.
Overall, it is clear that DCs are integral regulators of immunity. Indeed, as mentioned above, DCs integrate immune responses from a myriad immune cells and influence many aspects of both innate and adaptive immune response ( Fig. 9.5 ). Future studies will help determine the mechanism of how DCs can be best manipulated to harness the strongest immune response with least undesirable side effects.
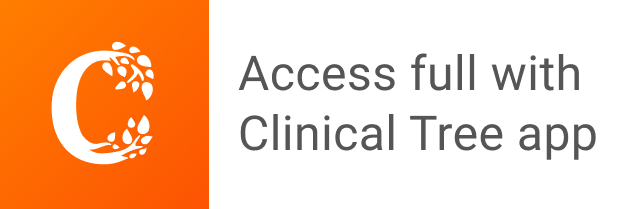