Contemporary Concepts in Soft-Tissue Wound Management
Andrew R. Evans, MD, FACS
Roman A. Hayda, MD, COL(Ret.)
Dr. Evans or an immediate family member is a member of a speakers’ bureau or has made paid presentations on behalf of Synthes; serves as a paid consultant to or is an employee of Zimmer; and serves as a board member, owner, officer, or committee member of the Center for Orthopedic Trauma Advancement (COTA) – BOD and the Orthopaedic Trauma Association. Dr. Hayda or an immediate family member is a member of a speakers’ bureau or has made paid presentations on behalf of AONA and Synthes; serves as an unpaid consultant to BioIntraface; and serves as a board member, owner, officer, or committee member of the American Academy of Orthopaedic Surgeons, METRC, and the Orthopaedic Trauma Association.
Keywords: healing; management; soft tissue; wound
INTRODUCTION
Musculoskeletal soft-tissue injury refers to mechanical, thermal, or chemical injury of skin, subcutaneous tissue, muscle, ligament, tendon, vascular, lymphatic, or periosteal tissues of the body (Figure 1). Due to their highly specialized structure and repair mechanisms, injury of tissues such as ligaments and tendons will be discussed in separate dedicated chapters of this book.
Skin tissue consists of stratified squamous (keratinized) epithelium, made up of an outer keratin layer, a series of layers transitioning from flattened squamous epithelial cells to cuboidal epithelial cells situated adjacent to a basal/basement membrane/lamina. Epithelium is avascular and is supplied nutrients and oxygen through diffusion from capillaries in the underlying basal lamina.
The primary function of skin tissue is to provide a water-impermeable barrier that resists mechanical abrasion/laceration and microbial invasion. When the epidermis is injured, the dermis and other underlying tissues are no longer contained in their native physical and chemical milieu and are therefore no longer protected from dessication, infection, and exposure to other cytotoxic conditions and/or chemicals.
Tissues underlying skin consist of a variety of connective tissues derived from undifferentiated mesenchymal stem cells. These tissue/cell types include adipose (adipocytes), fascia (fibroblasts), muscle (myoblasts/myocytes), vascular (endothelial cells), cartilage (chondrocytes), and bone (osteoblasts/osteocytes). This discussion of normal and injured soft tissues will focus primarily on the soft-tissue components including adipose, fascia, muscle, and vascular tissue. Obviously, these soft tissues do not live isolated from bone and cartilage, and we will discuss how the soft tissues are affected by the integrity and mechanical stability afforded by the underlying skeleton.
The structure of skeletal muscle consists of several hundred myoblasts lined up and fused end-to-end to form myotubes. Myotubes are filled with myofibrils consisting of myofilament arrays, the protein units responsible for the contractile function of skeletal muscle. Skeletal muscle fibers are arranged in parallel with intervening continuous capillaries, with individual myotubes contained in endomysium, muscle fascicles contained in perimysium, and entire muscles contained in epimysium. The microscopic structure and function of skeletal muscle will be described in further detail in other chapters. However, a facile understanding of this structure and function is required to understand the mechanisms for healing of skeletal muscle and its potential for regeneration. Skeletal muscle cells do not have mitotic capability, but skeletal muscle tissue does have regenerative capability due to the presence of
satellite, or mesenchymal stem cells. As a result, skeletal muscle is capable of undergoing hyperplasia (cell multiplication) and hypertrophy (cell fusion and spatial growth); however, the specificity and reliability of regenerating myoneural junctions is less well controlled or understood in response to injury.
satellite, or mesenchymal stem cells. As a result, skeletal muscle is capable of undergoing hyperplasia (cell multiplication) and hypertrophy (cell fusion and spatial growth); however, the specificity and reliability of regenerating myoneural junctions is less well controlled or understood in response to injury.
CELLULAR AND MOLECULAR MECHANISMS OF SOFT-TISSUE HEALING
Healing of skin, subcutaneous, fascial/connective, and muscle tissues often occurs through scar formation1 (Figure 1). Although each of these tissues has some potential for regeneration with “scarless” healing through stem cells, most wounds that result from trauma rely on scar tissue formation and even wound contracture. Advanced stem cell therapies that are being developed in the translational arena will be discussed in other parts of this chapter and textbook.
Soft-tissue wound healing occurs along a spectrum, with “scarless” tissue regeneration on one end, normal scar tissue formation in the center, and hypertrophic scar or even keloid formation on the other end (Figure 2). Wound healing classically occurs in three overlapping stages: inflammation, proliferation, and remodeling (Figure 3). The inflammatory phase of cellular recruitment to injury through autocrine and paracrine mechanisms typically lasts 48 to 72 hours following injury. The proliferative phase, where cell-mediated wound débridement occurs, granulation tissue forms, and vascularity proliferates, evolves over a period of at least 2 to 3 weeks, depending upon wound size and conditions. Once sufficient wound débridement has occurred to establish a well-vascularized wound bed, then the process of reepithelialization can occur, and eventually collagen fibrils are laid down, initially in a disorganized proliferative fashion, but are later gradually remodeled into a more organized collagen structure that never reaches the full tensile strength of normal tissue, but restores sufficient tissue integrity to reestablish the local biological environment.
Following the destruction or laceration of soft tissue, a hematoma containing fibrin and platelets forms. This hematoma facilitates hemostasis and also initiates a cellular and molecular cascade of events that result in the induction of an inflammatory response. That inflammatory response consists of degranulating platelets that release several inflammatory mediators (eg, platelet-derived growth factor [PDGF]) and cytokines (eg, interleukin-8 [IL-8]). Resident mast cells in the local tissues also release mediators including thrombin, complement factors, and others that increase blood flow through vasodilation and enhanced vascular permeability. The chemoattractants generated by this process recruit neutrophils and macrophages into the wound to phagocytose cellular debris, foreign debris, and bacteria. In response, neutrophils amplify the inflammatory reaction through release of inflammatory cytokines including IL-1, IL-6, and tumor necrosis factor (TNF)-α. These, along with transforming growth factor (TGF)-β, monocyte chemoattractant protein-1 (MCP-1), and various extracellular matrix (ECM) proteins further attract monocytes to the wound and stimulate differentiation into macrophages, which become the dominant cell type in the wound during the latter portions of the inflammatory phase, and subsequent transition to the proliferative phase of healing. This transition is mediated in large
part by macrophage-mediated release of chemicals including PDGF and vascular endothelial growth factor (VEGF), fibroblast growth factor (FGF), nerve growth factor (NGF), TGF-β, connective tissue growth factor (CTGF), and cysteine-rich 61 (Cyr-61), which promote the formation of proliferative granulation tissue. T-lymphocytes, fibrocytes, and eosinophils also participate in the inflammatory phase.
part by macrophage-mediated release of chemicals including PDGF and vascular endothelial growth factor (VEGF), fibroblast growth factor (FGF), nerve growth factor (NGF), TGF-β, connective tissue growth factor (CTGF), and cysteine-rich 61 (Cyr-61), which promote the formation of proliferative granulation tissue. T-lymphocytes, fibrocytes, and eosinophils also participate in the inflammatory phase.
The proliferative phase of wound healing, when granulation tissue is formed, is dominated from a cellular perspective by fibroblasts which form extracellular matrix (ECM), and endothelial cells which form blood vessels. Fibroblasts are stimulated to migrate in large part by PDGF, and upon arrival at the wound, many differentiate into myofibroblasts. Myofibroblasts are able to upregulate myocardin-related transcription factor-A, which facilitates expression of smooth muscle actin and causes wound contraction. Fibroblasts that remain travel along connective tissue fibers, aided by interactions between cell-surface integrins and ECM components
including fibrin, fibronectin, vitronectin, and hyaluronic acid. They clear pathways within wounded tissue to move through secretion of matrix metalloproteinases (MMPs) that not only break down ECM components to facilitate fibroblast migration, but also to facilitate new blood vessel ingrowth. Local endothelial cells break down the basement membranes of the capillaries they line to enhance vascular permeability and form tubules that eventually become new capillaries. Angiogenesis is promoted by secretion of VEGF, FGF, angiopoietin, TGF-β, as well as local hypoxia with lactic acid. As granulation tissue proliferates to cover the injured tissue/wound bed, the fibroblasts secrete cytokines such as Neu differentiation factor (NDF), which promotes keratinocyte migration from the wound edge and nearby hair follicles, thereby facilitating the process of epithelialization.
including fibrin, fibronectin, vitronectin, and hyaluronic acid. They clear pathways within wounded tissue to move through secretion of matrix metalloproteinases (MMPs) that not only break down ECM components to facilitate fibroblast migration, but also to facilitate new blood vessel ingrowth. Local endothelial cells break down the basement membranes of the capillaries they line to enhance vascular permeability and form tubules that eventually become new capillaries. Angiogenesis is promoted by secretion of VEGF, FGF, angiopoietin, TGF-β, as well as local hypoxia with lactic acid. As granulation tissue proliferates to cover the injured tissue/wound bed, the fibroblasts secrete cytokines such as Neu differentiation factor (NDF), which promotes keratinocyte migration from the wound edge and nearby hair follicles, thereby facilitating the process of epithelialization.
Once a wound is epithelialized, the scar tissue formed over this wound largely consists of disorganized type III collagen fibrils and ECM without any dermal appendages (eg, hair follicles, sweat glands), and epithelium. Many of the cells involved in the repair process, including fibroblasts, macrophages, and endothelial cells, go on to apoptosis. As the type III collagen fibrils are remodeled and reorganized into type I collagen, the strength of the wound repair improves to approximately 70% of its original tensile strength, and the scar becomes more flat, firm, and less vascular. A mature scar is made up of 80% to 90% type I collagen arranged in parallel bundles, the rest type III collagen. Collagen in normal skin is present in a basketweave orientation, improving its tensile strength over the parallel bundles of collagen in scar tissue. Scar is also thinner than normal skin in part due to the absence of dermal appendages and rete pegs that normally penetrate the dermis, as well as a lack of stem cells that typically inhabit these structures. The ECM of scar tissue also contains less elastin than normal skin, thereby reducing its elasticity, and when coupled with scar contracture mediated by fibroblasts and myofibroblasts, and significantly restrict the mobility of the injured soft tissues to the point where normal surrounding tissues such as muscles, tendons, and even joints no longer retain the mobility they had prior to the injury.
ACUTE SOFT-TISSUE INJURY PATTERNS
Laceration of soft-tissues refers to the incision or disruption of soft tissues in a way that exposes tissues at variable levels to the environment. Those tissues are no longer fully contained or protected within the sterile and homeostatic environment of the body. Superficial lacerations may only involve skin or subcutaneous tissues, whereas deeper lacerations can potentially involve all soft-tissue layers including muscle, fascia, ligaments, tendons, periosteum, and even bone in the case of open fractures. The tissue layers involved are disrupted at the cellular level, where cells are directly damaged and caused to undergo apoptosis and/or senescence. The mechanical environment in which the tissues typically live is disrupted through wound gaping or even flap formation. More profound alteration of the mechanical environment occurs when the underlying fascial, or even bony structures are disrupted, in which case ongoing mechanical injury to the tissues can occur until those tissues can be stabilized mechanically through skeletal fixation and suture repair. In addition to mechanical disruption and direct injury at the cellular level, lacerated tissues also experience disruption of their blood supply, thereby depriving variable tissue regions of nutrients and oxygen delivery. This lack of blood supply leads to a disruption of the normal respiratory process at the cellular level, resulting in ischemia and tissue necrosis unless that blood supply can be restored in a timely fashion.
Blood supply to and from tissues at all layers depend upon the integrity of the arterial, venous, and capillary networks that exist throughout the body. Laceration and most other forms of soft-tissue injury result in injury or compromise of those important vascular structures to some extent. Superficial tissues such as skin and subcutaneous tissue depend upon perforator vessels and capillary networks for their perfusion, and when those networks are damaged without sufficient collateral flow to compensate, skin necrosis occurs.
Most superficially, blisters of the skin can occur due to thermal, chemical, or mechanical injury at the interface of the epidermis and dermis. Injury at this level results in the accumulation of serous (nonhemorrhagic) or serosanguinous (hemorrhagic) fluid at the junction between the dermis and epidermis. Blistering of the skin from mechanical causes results from shearing forces being applied to the skin, whether it is superficial shearing forces coming from outside the body or shearing forces that emanate from deeper tissue disruptions, including fracture blisters.2 Blisters can occur in almost any area of the body; however, they occur significantly more commonly in the dorsal foot, ankle, lower leg, wrist, and forearm when associated with underlying fractures. Hemorrhagic blisters tend to be associated with more severe injuries of the skin and underlying tissues than nonhemorrhagic blisters, although this is not a universal finding. Hemorrhagic blisters are associated with more scarring than nonhemorrhagic blisters, significantly affecting cosmetic outcomes and patient satisfaction scores. The presence of fracture blisters, either hemorrhagic or nonhemorrhagic, is associated with an increased risk of skin breakdown and subsequent infection, irrespective of whether or not those blistered areas are incorporated into a surgical incision. Patients with diabetes mellitus and other conditions associated with peripheral vascular disease are at particularly high risk of soft-tissue complications and infection in blistered areas.
The Morel-Lavallee lesion (MLL), a traumatic separation of the hypodermis and underlying deep fascia resulting in
the development of a potential space that fills with blood, serosanguinous fluid, and necrotic fat, was first described by Victor-Auguste-Morel-Lavallee in 1863.3 The disruption of perforating vascular and lymphatic structures results in the formation of a hemolymphatic fluid collection containing blood clot, fibrin, as well as normal and necrotic fat—an environment at elevated potential risk of infection. Initially, when the shearing injury occurs between the superficial and deep tissue layers, a potential space is created that fills with blood, lymph, and fatty debris, much of which becomes necrotic due to the concomitant injury to tissue blood supply4 (Figure 4). Gradually, as this mixture of fluids is replaced by serosanguinous fluid, the volume of the collection typically increases, and eventually a pseudocapsule forms around it.5 Morel-Lavallee lesions have been described to occur in one of six patterns, based primarily on MRI imaging characteristics, as described by Mellado and Bencardino.6 Type 1 is a simple seroma, type 2 is a subacute hematoma, type 3 is a mature organized hematoma, type 4 is a closed fatty laceration complicated by perifascial dissection, type 5 is a perifascial nodular lesion, and type 6 is an infected lesion with sinus tract, septations, and capsular formation (Figure 5). If sufficient pressure is exerted on the overlying skin, the skin can experience necrosis, which ultimately leads to bacterial exposure and infection if débridement is not performed prior to recognition of this problem. Due to the necrotic and static nature of this fluid collection, it is often considered to be at risk of infection, even when overlying skin breakdown does not occur, and some studies have demonstrated that up to almost 50% of these lesions are culture positive when aspirated. Lesions treated with surgical débridement are at higher risk of infection than standard surgical wounds. Surgical indications, as well as techniques for management, remain controversial.7 Lesions treated with aspiration or surgical débridement are not only at higher risk of infection, but are also highly likely to recur with fluid reaccumulation. If closed lesions are treated nonsurgicallyy, and do not develop infection, the fluid pocket typically persists for months, and sometimes years. Typically the fluid will eventually resorb, and changes to the overlying skin will persist, including
thinning of the skin, which results in permanent cosmetic deformity at the site.
the development of a potential space that fills with blood, serosanguinous fluid, and necrotic fat, was first described by Victor-Auguste-Morel-Lavallee in 1863.3 The disruption of perforating vascular and lymphatic structures results in the formation of a hemolymphatic fluid collection containing blood clot, fibrin, as well as normal and necrotic fat—an environment at elevated potential risk of infection. Initially, when the shearing injury occurs between the superficial and deep tissue layers, a potential space is created that fills with blood, lymph, and fatty debris, much of which becomes necrotic due to the concomitant injury to tissue blood supply4 (Figure 4). Gradually, as this mixture of fluids is replaced by serosanguinous fluid, the volume of the collection typically increases, and eventually a pseudocapsule forms around it.5 Morel-Lavallee lesions have been described to occur in one of six patterns, based primarily on MRI imaging characteristics, as described by Mellado and Bencardino.6 Type 1 is a simple seroma, type 2 is a subacute hematoma, type 3 is a mature organized hematoma, type 4 is a closed fatty laceration complicated by perifascial dissection, type 5 is a perifascial nodular lesion, and type 6 is an infected lesion with sinus tract, septations, and capsular formation (Figure 5). If sufficient pressure is exerted on the overlying skin, the skin can experience necrosis, which ultimately leads to bacterial exposure and infection if débridement is not performed prior to recognition of this problem. Due to the necrotic and static nature of this fluid collection, it is often considered to be at risk of infection, even when overlying skin breakdown does not occur, and some studies have demonstrated that up to almost 50% of these lesions are culture positive when aspirated. Lesions treated with surgical débridement are at higher risk of infection than standard surgical wounds. Surgical indications, as well as techniques for management, remain controversial.7 Lesions treated with aspiration or surgical débridement are not only at higher risk of infection, but are also highly likely to recur with fluid reaccumulation. If closed lesions are treated nonsurgicallyy, and do not develop infection, the fluid pocket typically persists for months, and sometimes years. Typically the fluid will eventually resorb, and changes to the overlying skin will persist, including
thinning of the skin, which results in permanent cosmetic deformity at the site.
Direct pressure on skin and soft tissues, if sufficiently forceful or prolonged, induced ischemia and/or mechanical damage to that soft tissue that results in pressure-induced skin necrosis, or ulcer formation. These ulcers/wounds vary depending upon the depth of tissue involved and the surface area of those affected tissues. Pressure sores/ulcers are a significant source of morbidity and mortality, particularly in patients with impaired mobility, increased age, and with comorbidities that impair the normal wound healing process.
Ballistic injuries are a unique form of injury to the skin and soft tissues because of the area of injury peripheral to the primary site of injury. This is commonly seen in bullet wounds, where there is direct laceration or disruption of the tissues through which the bullet passes, but there is also a variable area of injury surrounding that bullet tract, caused by a traumatic wave of energy capable of disrupting the viability of tissues surrounding that area. Higher velocity firearms, larger bullet sizes and postimpact shapes (ie, flattening of hollow-tipped bullets), and closer range injury all lead to higher levels tissue damage, including ballistic injury of tissues surrounding the primary point(s) of penetration. Blast effects, resulting from explosions, occur in similar fashion to ballistic injuries described above, but on a much larger scale, potentially resulting in catastrophic soft-tissue injury or even amputation.
Thermal and chemical burns are additional forms of soft-tissue injury that can range from superficial to deep. They can affect all layers of soft tissues, and typically result in necrosis of the layers they affect.8 There is typically a focus of injury where the primary injury occurs, and a peripheral area of lesser injury until reaching tissues that are unaffected. Patients who experience thermal or chemical burns are often left with scarring, often severe, and therefore can experience contractures that prohibit normal motion of the affected skin and soft tissues. When burns occur in areas overlying joints, contractures often develop in ways that inhibit normal joint motion, and can require contracture releases.
Lacerations resulting in the exposure of underlying osseous fractures are referred to as open or compound fractures. The presence of the underlying fracture creates a unique clinical situation in which the soft tissues, extending down to and including the bone, are at elevated risk of infection. The underlying skeletal instability is harmful to the overlying soft tissues, thereby creating an area of variably compromised soft-tissue viability at all layers, further enhancing the risk of infection to this area. Open fractures typically require timely surgical débridement and skeletal stabilization to minimize the risk of infection, or even amputation. The severity of open fractures has been graded and classified many ways over the last several decades; however, the two most prominent classifications currently in use include the Gustilo and Anderson classification, and the Orthopaedic Trauma Association (OTA) Open Fracture Classification (OFC).9,10 The Gustilo and Anderson classification rates the severity of injury based upon the size and depth of soft-tissue injury, the extent of fracture comminution and instability of the fracture pattern, and the nature and degree of contamination at the site of injury. The Gustilo and Anderson classification, although arguably the most commonly used classification in clinical practice among orthopaedic and nonorthopaedic specialties, suffers from a well-documented lack of interobserver reliability. As a result, the OTA OFC was created using methods that clearly demonstrate significantly enhanced interobserver reliability over Gustilo and Anderson. It also helps to stratify injury severity using a distinct nomenclature and scoring system, aids in the development of treatment strategies, and also possesses the capability to predict or anticipate outcomes for patients who sustain open fractures. The OTA OFC incorporates into its nomenclature and structure, injury severity stratifications for skin, muscle, and bone, as well as accounts for whether any associated arterial injury creates limb ischemia, and how extensive any wound contamination is around the open fracture. This classification helps to predict whether certain injury patterns, or levels of severity, will require multiple surgical debridements, benefit from use of modalities such as negative pressure wound therapy (NPWT) vacuum-assisted closure (VAC) devices or antibiotic-impregnated poly methyl methacrylate (AIPMMA) beads, and what levels of injury severity are likely to lead to the need for amputation.
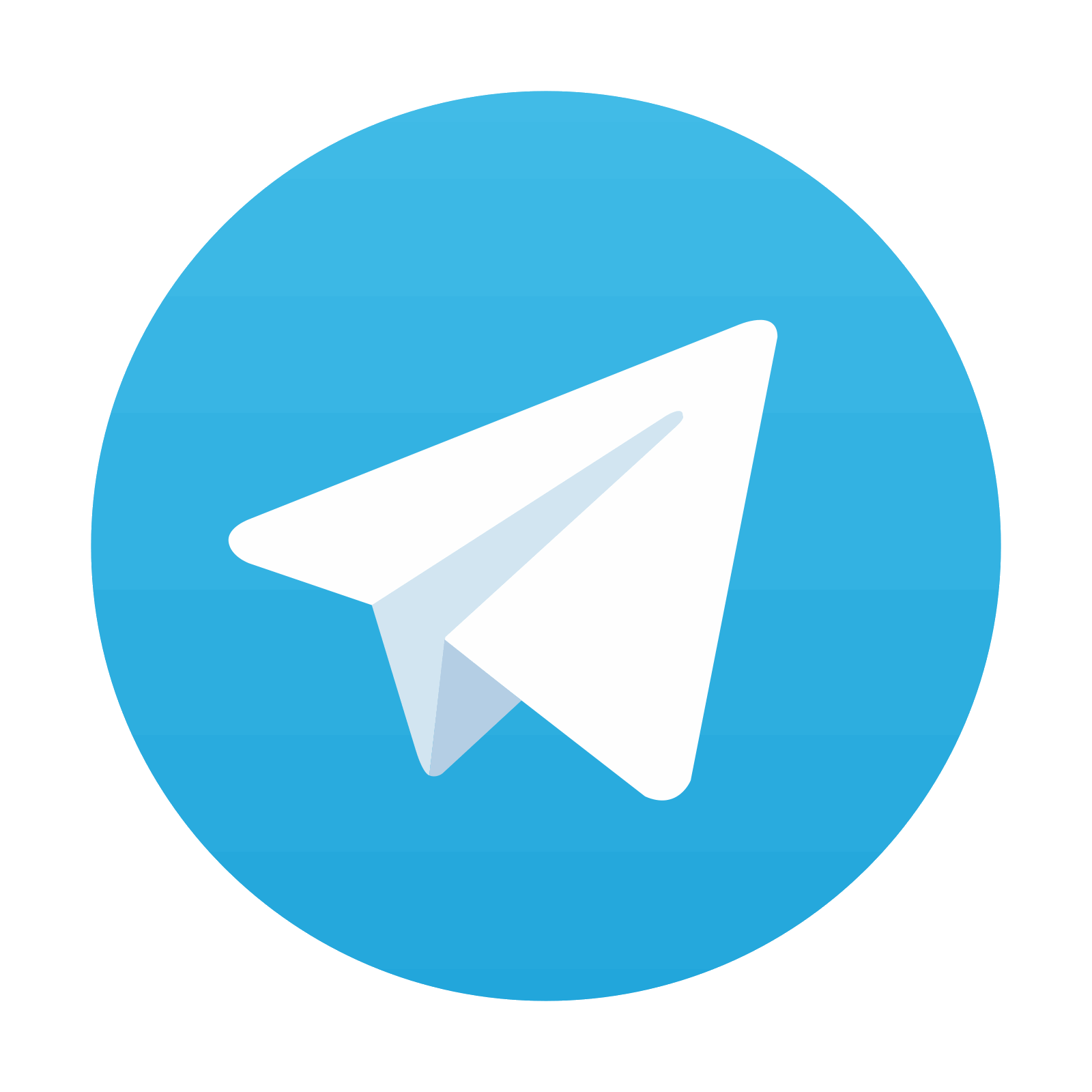
Stay updated, free articles. Join our Telegram channel
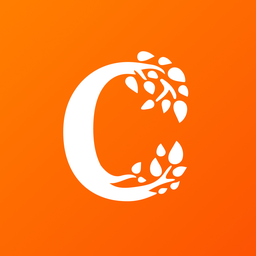
Full access? Get Clinical Tree
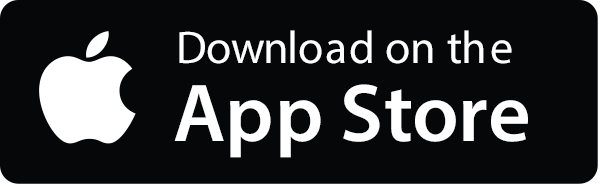
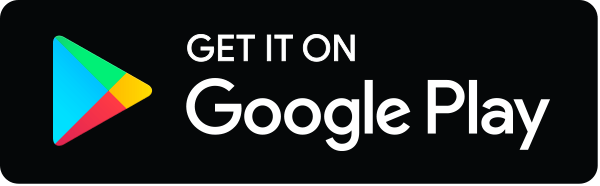