Introduction
The congenital myopathies are a clinically, genetically and pathologically heterogeneous group of muscle disorders defined in many patients by the presence of particular histopathological features. They emerged as a group of disorders with the wider application of histochemistry and electron microscopy in the 1950s and 1960s, when abnormal structural defects were identified in association with a particular phenotype, before any molecular causes were known. Historically, the recognition of this group of disorders probably dates from the description of ‘a new congenital non-progressive myopathy’ by , later named central core disease (see below), and the subsequent demonstration of the striking histochemical picture by . Presentation of congenital myopathies is often at birth or in early childhood, but some cases with a structural defect may have adult onset and are thus not strictly ‘congenital’. Some of these are part of a clinical spectra, whereas others are different entities sharing pathological features with congenital cases. Clinically, the congenital myopathies often fall into the ‘floppy infant’ category with a variable degree of hypotonia ( ). Muscle weakness is often, but not always, relatively non-progressive, particularly in early decades, but diaphragmatic weakness and respiratory insufficiency may be disproportionate. Structural abnormalities in the central nervous system or peripheral nerves are absent, and congenital myopathies are therefore regarded as primary myopathies.
Advances in molecular analysis and the wide application of next-generation sequencing and gene panels have led to the identification of several causative genes and a wider appreciation of the clinical phenotype and morphological features associated with them ( ). Some of the more common forms are relatively well-defined disorders with a well-recognized pathological feature, while others are very rare and may be based on very few isolated patients. In these, it is not yet clear if all are distinct genetic entities. The genes responsible for several congenital myopathies described before the molecular era have now been identified, and it is apparent that they often form part of a clinicopathological spectrum rather than being distinct disease entities. For example, the presence of cap-like areas is associated with several genes (see below); zebra body myopathy is caused by a mutation in the ACTA1 gene encoding skeletal actin, sarcotubular myopathy by the gene for TRIM32, spheroid myopathy by the MYOT gene encoding myotilin and reducing body myopathy by the gene for four-and-half LIM domain 1 ( FHL1 ) ( ). All of these genes are also associated with other phenotypes. Few of the pathological features are specific for a particular disorder. It is now apparent that there is considerable pathological overlap between the various congenital myopathies, and the pathological distinction between them is not always clear. Mutations in different genes can lead to the presence of the same histopathological feature, sometimes as a result of functional association of the gene products, and mutations in the same gene can give rise to a variable clinical and pathological phenotype, some of which may be classified in other groups of disorders ( Tables 15.1 and 15.2 ). This has challenged the traditional classification of several disorders. The pathogenic mechanisms underlying the presence of the structural abnormalities are not fully understood, although various pathways have been considered ( ).
RODS ACTA1, NEB, TPM2, TPM3, TNNT1, CFL2, KBTBD13, KLHKL40, KLHL41, LMOD3, MYPN, TNNT3, MYOB18, TTN, RYR1, RYR3, EXOSC3, PPA2 CORES OF VARYING SIZE RYR1, SELENON (formerly SEPN1), ACTA1, KBTBD13, TTN, MYH7, CFL2, MYH2, ACTN2, MEGF10 and others CENTRAL NUCLEI
ACTA1, RYR1, NEB, CFL2, KBTBD13 RODS AND/OR CAPS TPM2, TPM3, ACTA1, TTN FIBRE TYPE DISPROPORTION WITH NO OTHER DEFECT ACTA1, TPM2, TPM3, SELENON, RYR1, HACD1 ACTIN ACCUMULATION ACTA1, CFL2 |
Gene | Inheritance | Protein | |
---|---|---|---|
Nemaline Myopathies | |||
ACTA1 | AD (AR) | Skeletal α-actin | |
NEB | AR | Nebulin | |
TPM3 | AD | α-Tropomyosin, slow | |
TPM2 | AD | β-Tropomyosin | |
TNNT1 | AR | Slow troponin T1 | |
TNNT3 | AR | Fast troponin T3 | |
CFL2 | AR | Cofilin-2 | |
KBTBD13 | AD | Kelch repeat- and btb/poz domain-containing protein 13 | |
KLHL40 | AR | Kelch-like family member 40 | |
KLHL41 | AR | Kelch-like family member 41 | |
LMOD3 | AR | Leiomodin 3 | |
MYOB18 ∗ | AR | Myosin XVIIIB | |
Other Myopathies with Rods | |||
Rods and cores | RYR1 | AR or AD | Ryanodine receptor 1 |
ACTA1 | AD (AR) | Skeletal α-actin | |
NEB | AR | Nebulin | |
Rods and caps | TPM2 | AD | β-Tropomyosin |
TPM3 | AD (AR) | α-Tropomyosin, slow | |
ACTA1 | AD | Skeletal α-actin | |
TTN | AR | Titin | |
Congenital Myopathies with Cores | |||
Central core disease | RYR1 | AD or AR | Ryanodine receptor 1 |
Multi-minicore disease | SELENON (SEPN1) | AR | Selenoprotein N |
Congenital myopathy with or without cardiomyopathy | TTN | AR | Titin |
Central Nuclear Myopathies | |||
Myotubular myopathy | MTM1 | XLR | Myotubularin |
Centronuclear myopathy | DNM2 | AD (AR) | Dynamin-2 |
BIN1 | AR | Amphiphysin-2 | |
RYR1 | AD, AR | Ryanodine receptor 1 | |
SPEG | AR | SPEG complex locus | |
Centronuclear myopathy with cores | CCDC78 | AD | Coiled-coil domain-containing protein 78 |
Congenital myopathy with or without cardiomyopathy | TTN | AR | Titin |
Surplus Protein Congenital Myopathies | |||
Actin aggregation | ACTA1 CFL2 | AD AR | Skeletal α-actin Cofilin-2 |
Zebra body myopathy | ACTA1 | ?AD | Skeletal α-actin |
Myosin storage myopathy | MYH7 | AD | Slow/β-cardiac myosin heavy chain |
Myopathies with caps | TPM2 | AD | β-Tropomyosin |
TPM3 | AD | α-Tropomyosin, slow | |
ACTA1 | AD | Skeletal α-actin | |
TTN | AR | Titin | |
Reducing body myopathy ♦ | FHL1 | XLD | Four-and-half LIM domain-1 protein |
Spheroid body myopathy ♦ | MYOT | AD | Myotilin |
FLNC | AD | Filamin C | |
Myopathies with Abnormal Type 2A Fibres | |||
Myopathy with congenital joint contractures and ophthalmoplegia | MYH2 | AD | Myosin heavy chain IIa |
Myopathy with absent/abnormal type 2A fibres and ophthalmoplegia | MYH2 | AR | Myosin heavy chain Iia |
Congenital Fibre Type Disproportion | |||
ACTA1 | AD | Skeletal α-actin | |
SELENON | AR | Selenoprotein N1 | |
TPM3 | AD | α-Tropomyosin, slow | |
TPM2 | AD | ||
RYR1 | AD or AR | Ryanodine receptor | |
MYH7 | AD | Myosin heavy chain 7 | |
HACD1 (PTPLA) | AR | 3-Hydroxyacyl-CoA dehydratase 1 |
∗ Mutations in MYOD18 have only been identified in a few patients with a complex phenotype that is not typical of nemaline myopathy.
♦ These disorders were historically classified as congenital myopathies but now are usually grouped with other disorders (see text).
Inheritance of congenital myopathies may be autosomal recessive, autosomal dominant or X-linked, and there is a high incidence of de novo dominant mutations.
In this chapter we describe the pathology of the more common congenital myopathies with particular structural features that a pathologist is likely to encounter. In addition, we also describe some of the more recently identified entities. We have deliberately steered away from a molecular categorization, as it is the pathology in association with the clinical phenotype that usually leads to identification of the causative gene defect. Molecular advances, however, have led to greater knowledge of the range of morphological features that can occur in association with a broad range of clinical phenotypes and molecular defects. We include in this chapter a group of disorders caused by mutations in genes that encode sarcomeric proteins but that do not always show a particular structural pathological feature. Some of these disorders present with congenital arthrogryposis, a clinical feature of several syndromes and not a diagnostic feature, and a myopathic aetiology, and muscle weakness may not be as apparent as in cases with structural abnormalities. Some of the same genes, however, can result in a structural defect or present in adulthood, but the disorder is still classified with congenital myopathies (see Table 15.2 ), emphasizing the pathological and genetic overlap. The most common congenital myopathies are the core myopathies (central core disease and multi-minicore myopathy), nemaline myopathies, and myotubular and centronuclear myopathies (see below; see Table 15.2 ) ( ), but pathological overlap is now recognized. Additional rare disorders characterized by other morphological features are listed in Table 15.3 .
Gene | Inheritance | Protein | Function of Protein |
---|---|---|---|
CACNAIS ∗ | AD or AR | Calcium voltage-gated channel subunit alpha1 S | Excitation-coupling |
SCN4A ∗∗ | AR | Sodium voltage-gated channel alpha subunit 4 | Ion-channel |
STAC3 | AR | SH3 and cysteine-rich domains 3 | Excitation-coupling |
TRDN | AR | Triadin | Excitation-coupling |
ZAK | AR | Mitogen-activated protein triple kinase | Signal transduction |
MEGF10 | AR | Multiple epidermal growth factor (EGF)-like domains 10 | Satellite cell function |
PYROXD1 | AR | Pyridine nucleotide-disulphide oxidoreductase domain 1 | Regulation of redox potentials |
MYMK (TMEM8C) | AR | Myomaker | Transmembrane protein involved in fusion of myoblasts |
MYL1 | AR | Fast myosin light chain 1 | Alkali light chain involved with muscle contraction |
TRIP4 | AR | Activating signal cointegrator-1 | Transcription coactivator |
UNC45B | AD or AR | Uncordinated mutant number-45 myosin chaparone B | Myosin assembly and lens development |
∗ See Chapter 20 for involvement in other disorders.
∗∗ See Chapter 21 for involvement in other disorders.
Myopathies with Structural Defects
In this section we discuss the disorders that have traditionally been classified as ‘congenital myopathies’, core myopathies, nemaline myopathies and centronuclear myopathies, although it is now recognized that there is considerable pathological overlap among them.
Overview of Clinical Features
The spectrum of clinical features, severity and progression of disease is variable in the different forms of congenital myopathy ( ). Hypotonia is usually present at birth or early infancy, although rare cases with hypertonia and a ‘stiff’ phenotype have been reported ( ). Several additional clinical features also distinguish the various congenital myopathies. Muscle weakness may be predominantly proximal and of limb-girdle distribution, thus resembling muscular dystrophy or mild forms of spinal muscular atrophy, or it may be more generalized. In some individuals, weakness may show marked involvement of the axial muscles and the face, and a few may show prominent distal involvement. A long ‘myopathic’ face is a common feature, particularly in nemaline myopathy, and extraocular involvement occurs in some forms. Structural abnormalities of the central nervous system or peripheral nerves do not usually occur and intelligence is usually normal. Although generally non-progressive, diaphragmatic involvement may be disproportionate to overall muscle weakness, in particular in some cases of nemaline myopathy and multi-minicore disease (see also below MEGF10 ).
Arthrogryposis may be a feature in some severe cases of congenital myopathies ( ), as well as being a symptom in some disorders without structural defects (see below). Lordosis, spinal rigidity, scoliosis and joint laxity are common, and hip dislocation is a particular feature of RYR1- related core disease, although all of these can also be clinical features of other disorders: for example, Ullrich congenital muscular dystrophy.
Myasthenic-like symptoms can also be present in some cases with a congenital myopathy, with defects in various genes including TPM2 , TPM3, BIN1 , DNM2, MTM1 and RYR1 (see Ch. 21 and ). It is important to identify these individuals, as they often respond well to therapy.
Investigations
Serum creatine kinase (CK) levels are usually normal and electrophysiological studies rarely help in diagnosis but can be of use in patients with myasthenic-like symptoms. Ultrasound imaging often shows increased echo and may reveal differential involvement of muscles. This can be helpful in deciding which muscle to sample. The differential involvement of muscles is clearly seen with magnetic resonance imaging (MRI), and data indicate that particular patterns of selective involvement of thigh and lower leg muscles are associated with mutations in certain genes and are helpful in directing molecular analysis ( ). Muscle biopsy, with detailed histochemical studies, supplemented by immunohistochemistry and electron microscopy, is essential for the diagnosis of congenital myopathies for directing and for interpreting molecular analysis.
General Pathological Features of Congenital Myopathies
Atrophy or hypotrophy (small fibres that have not attained their normal diameter) of type 1 fibres is seen in several congenital myopathies, and may appear as fibre type disproportion (see below). There is often a marked predominance or uniformity of type 1 fibres, but the intensity of staining is often less than that seen in normal muscle and intermediate between type 1 and 2 fibres. The involvement of type 1 fibres, however, is not universal and in some cases it involves type 2 fibres (e.g. some cases of nemaline myopathy, see below). Antibodies to myosin isoforms confirm the slow phenotype of most fibres but, again, the intensity of labelling may be less than in normal muscle. In addition, co-expression of developmental isoforms and slow and/or fast myosin may occur in very young patients. Necrosis and regeneration are not typical features of congenital myopathies. Fibrosis is also rare but can occur (see section on core myopathies and nemaline myopathies). Scattered, very small fibres (< 5 μm) containing fetal myosin (sometimes colloquially referred to as ‘pinpricks’), however, are often seen (see Figs. 6.25 and 6.26), but the origin of these is not clear, nor whether they represent attempts at regeneration. Centrally placed nuclei are a particular feature of myotubular and centronuclear myopathies (see below), and they are also common in association with mutations in the RYR1 and TTN genes (see below). Multiple internal nuclei are a particular feature of patients with mutations in the PYRODX1 gene (see below).
Core Myopathies
Areas devoid of oxidative enzyme stains are the characteristic pathological feature of the ‘core myopathies’. However, there is considerable variability and overlap in the clinical, pathological features and genotypes that can make differential diagnosis difficult. We therefore find it useful to refer to this group of disorders with clinical features of a congenital myopathy collectively as ‘core myopathies’. We discuss, however, the two historical categories of central core disease and multi-minicore myopathy as these are well established terms but also discuss the expanding clinical, pathological features of the genotypes associated with cores of varying types.
Central Core Disease
Historically, the identification of ‘central core disease’ dates back to when described a ‘new congenital non-progressive myopathy’ affecting five patients in three generations of the same family, ranging in age from 2 to 65 years. The main clinical features were hypotonia and delay in motor milestones in infancy, and a mild non-progressive weakness, affecting proximal muscles more than distal, and the legs more than the arms. The muscle was characterized by amorphous-looking central areas within the muscle fibres, and subsequently suggested the name ‘central core disease’. A second case documented by was studied histochemically ( ) and the classical histochemical features noted, in particular well-delineated areas that ran down a considerable length of the fibres that were devoid of oxidative enzyme stain and phosphorylase. These core areas were not necessarily central and many fibres had multiple large cores. In addition, the normal fibre typing was lost and the fibres had a uniform appearance of only type 1 fibres. Electron microscopy showed a virtual absence of mitochondria and sarcoplasmic reticulum in the core region, a marked reduction in the intermyofibrillar space and an irregular pattern (streaming) of the Z lines ( ).
Since these early reports there have been many additional cases with this phenotype associated with prominent core lesions. With the advent of molecular genetics there has been a greater understanding of the clinical and pathological phenotype of central core disease as well as a greater understanding of the consequences of the genetic causes ( ).
The inheritance of many cases of central core disease is autosomal dominant, with variable penetrance, several of which are sporadic, de novo dominant cases. An increasing number of cases with recessive inheritance in the RYR1 gene as the cause of central core disease have also been identified. These often have more marked axial weakness, illustrating the overlap with multi-minicore myopathy (see below), and they may have ophthalmoplegia ( ).
Central core disease is one of the most common congenital myopathies ( ). Cases identified prior to the molecular era showed a fairly consistent clinical phenotype, presenting with hypotonia and developmental delay. The phenotype is now known to be wider, and cases with a severe presentation with features of the fetal akinesia sequence have been reported as well as several cases with recessive inheritance ( ). Many of the severely affected infants require ventilation at birth, with progression leading to death in infancy. Other cases, in contrast, may show considerable improvement, and it may be possible to wean the infants off tracheostomy ventilation; one reported child eventually became independently ambulant ( ).
Weakness in most familial cases is pronounced in the hip girdle and in the axial muscle groups and may be associated with muscle wasting. Facial involvement is usually mild and lack of complete eye closure may be the only finding. Orthopaedic complications are common and include congenital dislocation of the hips and scoliosis. Contractures, other than Achilles tendon tightness, are rare, and many affected individuals have marked ligamentous laxity, occasionally associated with patellar instability, illustrating the phenotypic overlap with disorders such as Ullrich congenital muscular dystrophy. Apart from the most severe neonatal cases, and some of those with congenital dislocation of the hips ( ), most patients achieve independent walking. The course of central core disease is often static, or only slowly progressive, even over prolonged periods of time ( ), although there may be progression with age. Primary cardiac involvement is rare and respiratory involvement is usually milder than in other congenital myopathies, except in the severe neonatal cases. Serum CK activity is usually normal or only mildly elevated. A striking feature of central core disease is the differential muscle involvement, which can be shown on muscle ultrasound and, more strikingly, with MRI of muscle which reveals a characteristic pattern of selective involvement, even within the quadriceps, with sparing of the rectus femoris ( ). This is helpful when selecting the site for a muscle biopsy as well as for diagnosis ( ).
The RYR1 gene that is responsible for both dominant and recessive forms of core myopathies is also associated with other phenotypes, including malignant hyperthermia susceptibility, King–Denborough syndrome and exercise intolerance with rhabdomyolysis (see Ch. 21 ; ). As the RYR1 gene is associated with malignant hyperthermia susceptibility, all patients with central core disease are considered at risk and appropriate precautions need to be taken.
Histopathology
Fibre size variation occurs but is often mild. Fibre hypertrophy is common, particularly in adults ( Fig. 15.1 ). When fibre typing is retained, the cores have a predilection for type 1 fibres but fibre type uniformity is common, with most fibres staining as type 1 fibres, with the associated properties of slow fibres (see Fig. 15.1 ). A few fibres may co-express fast myosin and there may be a population of very small fibres (‘pinpricks’ < 5 μm in diameter) with fetal myosin scattered throughout the biopsy (see Fig. 6.26 ). The intensity of stain of the type 1 fibres, however, may not always be as strong as in normal muscle. Classical cores may be central or peripheral, single or multiple, but clearly demarcated cores are not always evident in all cases (see Fig. 15.1 ) and some genetically proven cases only show an appearance of fibre type disproportion ( ) (see below). Very young cases, in particular, may only show type 1 uniformity or predominance, suggesting there is an age-related development of the cores (see Fig. 15.1 ; ). This is well illustrated in a mouse model in which an RYR1 mutation caused an age-related appearance of different size cores and ultimately led to the appearance of nemaline rods ( ). Other cases may show only subtle unevenness in oxidative enzyme stains ( Fig. 15.2 ) or multiple focal areas of disruption of variable size, resembling minicores, making a histopathological distinction difficult ( ) ( Fig. 15.1 ; see below). Recessive cases, in particular, may show multiple cores rather than prominent central cores ( ). It is important to remember that core formation is a secondary morphological consequence of disturbances in cellular events that may not itself be the reason for the muscle weakness ( ).


In many cases of central core disease, particularly dominantly inherited cases, the cores are of the ‘structured’ type, as they retain a striated myofibrillar pattern and myofibrillar adenosine triphosphatase (ATPase) activity, although myofibrils of the core are often very contracted ( Fig. 15.3 ). The distinction of ‘structured’ versus ‘unstructured’ cores is not of diagnostic value. In ‘unstructured cores’, myofibrillar ATPase activity is lost ( Fig. 15.4 ) and there is severe myofibrillar disruption with pronounced accumulation of smeared Z line material which may account for the red appearance reported with the Gomori trichrome ( ). The cores in recessive cases are often unstructured, and variable in size, affecting many or only a few sarcomeres; or they may even stretch across the width of a fibre ( ). The length of the cores can be variable but in dominantly inherited cases they are long, extending down an appreciable length of a fibre. The area devoid of mitochondria may be more extensive than the apparent ultrastructural myofibrillar disruption. Sarcoplasmic reticulum and T tubules may also be reduced in cores but some tubular structures may be apparent within them. Cores are often delineated by a rim of periodic acid–Schiff (PAS) stain but lack glycogen within them and consequently also lack phosphorylase (see Ch. 4 ). Immunohistochemistry shows that desmin may accumulate at their perimeter or within them ( Fig. 15.5 ), and other proteins that have been shown to accumulate in cores include αB-crystallin, filamin C, small heat-shock proteins, myotilin, RyR1 and DHPR ( ).



Internal nuclei had not been considered a feature of central core disease in studies of early cases but it is now appreciated that they can be an important indicator of central core disease and defects in the RYR1 gene ( Fig. 15.6a, d ). In some cases they may be numerous, and several may be in a central position, resembling a centronuclear myopathy ( ; see below), but cores may not be obvious. Similarly, an increase in connective tissue was not considered a feature of ‘classical’ cases but can occur, and in some samples there may also be extensive adipose tissue (see Fig. 15.6d ; Fig. 15.6e ). In these samples the separation of fascicles of fibres by adipose tissue and fibrous tissue may cause diagnostic confusion with a muscular dystrophy. Some of these samples may show only subtle unevenness of oxidative enzyme stains, while others show large classical cores or multiple small cores (minicores; see Fig. 15.6b, c, f ).

Although cores are the characteristic feature of central core disease caused by mutations in the gene encoding the ryanodine receptor 1 ( RYR1 ), core formation can also occur following tenotomy, following neurogenic atrophy (see Ch. 9 ). Small focal cores (minicores) can be associated with several gene defects, including SELENON (formerly SEPN1 ) and MYH2 (see below) and the ACTA1, MYH7, TTN, CFL2, KBTBD13, ACTN2 and MEGF10 genes, encoding skeletal α-actin, β-myosin heavy-chain, titin, cofilin2, a protein of the Kelch family, α-actinin 2, and multiple epidermal growth factor-like domains 10, respectively (see Table 15.1 and sections below; ). Cores in association with central nuclei have also been identified in association with the CCDC78 gene ( ). Cores can coexist with rods ( Fig. 15.7 ) and be associated with RYR1, ACTA1 and NEB mutations (see below) ( ). If rods are in a focal aggregate this may appear as a core, as there are no mitochondria and thus no oxidative enzyme stain. In cases described as ‘core-rod myopathy’ the cores and rods are in separate fibres. In some cases with RYR1 mutations, only a few fibres may show rods ( Fig. 15.8 ; ). The coexistence of rods and cores is likely to be genetically heterogeneous as there are examples of cases where linkage to RYR1 and to the loci of nemaline myopathy (see below) has been excluded.


Molecular Genetics
Central core disease is caused by mutations in the gene for the skeletal muscle ryanodine receptor ( RYR1 ) on chromosome 19q. The same gene is also responsible for malignant hyperthermia susceptibility (although additional loci are also linked to this), King–Denborough syndrome and exercise intolerance associated with rhabdomyolysis. The precise association between central core disease and malignant hyperthermia is not clear but, as pointed out previously, all patients with central core disease are considered at risk and appropriate precautions need to be taken.
The RYR1 gene contains 106 exons and encodes the skeletal muscle ryanodine receptor protein (RyR1), named after the fact that it binds ryanodine. The receptor is a large transmembrane, tetrameric structure of the sarcoplasmic reticulum and is involved in the regulation of cytosolic calcium levels and excitation–contraction coupling. Genotype–phenotype correlations suggest that mutations in the cytoplasmic N-terminal domain and the cytoplasmic central domain mostly result in malignant hyperthermia susceptibility rather than in central core disease, while mutations affecting the C-terminal exons of the gene commonly result in central core disease ( ). Mutations in the C-terminal exons (95–102) often result in the classical central core pathology with large cores and account for approximately 66% of cases. Recessive mutations can occur in any region of the gene and are often associated with multiple minicores ( ). The majority of mutations in the RYR1 gene are missense mutations, although deletions have also been detected ( ).
The large size of the RYR1 gene makes molecular analysis laborious, although there are ‘hot spots’ for mutations that can help direct analysis ( ). The phenotypic and histopathological variability associated with the RYR1 gene is broad, but the use of gene panels and next-generation sequencing has considerably assisted diagnosis and the identification of mutations. There are, however, many polymorphisms and variants of unknown significance in this large gene. Studies of the RyR1 protein by immunoblot analysis can reveal a reduction and help to determine if a change is pathogenic ( ). Another complicating factor in the RYR1 gene is that tissue-specific epigenetic silencing of the normal allele, resulting in expression of only the mutant allele, may occur ( ). Although further studies of the cases reported by Zhou et al revealed an additional mutation in four out of the five cases, casting doubt on the proposal of epigenetic silencing, gene silencing could not be excluded in the fifth ( ). This study of Zhou et al highlights the limitation of only studying genomic DNA and the contribution of genetic modifiers is now appreciated ( ).
In summary, the clinical and histopathological spectra associated with mutations in the RYR1 gene are wide. The use of the term ‘central core’ disease for all of them may be confusing, as some biopsies do not show classical cores. They are linked, however, by common clinical features, and similar patterns of muscle involvement, indicating a spectrum of one disorder. For the histopathologist, difficulties in diagnosis may arise if the classical features are not present and because of the histopathological overlap between the various congenital myopathies. In our experience mutations in the RYR1 gene are particularly common and digenic problems should therefore sometimes be considered, particularly if the phenotype is atypical ( ). The pathology associated with one gene can be masked by that of the other ( ). The features that should alert the pathologist to defects in the RYR1 gene are central nuclei, any unevenness in oxidative enzyme stain, be it marked or subtle, and type 1 fibre uniformity or marked predominance. When fibre type disproportion in the absence of cores is present, the RYR1 gene also has to be considered. The coexistence of cores and rods in any fibres also suggests an RYR1 mutation should be considered but can also occur in association with other gene defects such as nebulin and skeletal α-actin (see below).
Multi-minicore Myopathy
In documented two unrelated children with a benign congenital non-progressive myopathy associated with multifocal areas of degeneration in the muscle fibres and suggested the name ‘multicore disease’. Since the original description, there have been several reports of cases with a wide range of clinical phenotypes associated with similar histopathological features. The defining histopathological feature is multiple small areas devoid of oxidative enzymes which lack mitochondria and, ultrastructurally, show focal disruption of the sarcomeric pattern.
The clinical features of cases with multicores are variable and multiple cores can occur in association with mutations in several genes (see sections above and below), and to some extent are a non-specific pathological feature that can be seen in many disorders. Correlation with clinical phenotype is thus essential. Multi-minicore myopathy is not a single entity and is a name that has been given to several myopathies in which a muscle biopsy shows multifocal areas devoid of oxidative enzyme stains. They could therefore be described more accurately as ‘myopathies with multi-minicores’.
Histopathology
The defining feature is multiple focal areas of myofibrillar disruption that lack mitochondria. These can be demonstrated with the oxidative enzyme stains reduced nicotinamide adenine dinucleotide-tetrazolium reductase (NADH-TR), cytochrome c oxidase (COX) and succinate dehydrogenase (SDH) and may appear as punctate or more diffuse areas devoid of stain ( Fig. 15.9 ). The NADH-TR reaction is less specific for absent mitochondria, as unevenness of stain can also relate to general disruption of ultrastructure and loss of myofibrils. Assessment with COX or SDH should therefore also be performed. In some cases, the unevenness of oxidative stain may be subtle and difficult to define as abnormal. Ultrastructurally, minicores show a variable degree of focal disruption of myofibrils that affects only a few sarcomeres. In some fibres, only misalignment of the myofibrils compared with surrounding myofibrils may be seen; in others, focal Z line streaming may occur, while in others the normal sarcomeric structure may be completely disrupted in a varying number of sarcomeres (see Ch. 5 ). Small focal areas adjacent to capillaries resembling minicores are a common non-specific feature. The consistent feature of minicores, however, is the absence of mitochondria, which may extend over a greater area than the disrupted myofibrils. Detailed ultrastructural studies are needed to observe this.

As in central core disease, immunocytochemistry can be helpful in observing the lesions, and proteins such as desmin, myotilin and filamin C can accumulate within them ( ), but there is no specific histopathological method to distinguish the molecular origin of any cores. In contrast to some central cores, minicores are not usually delineated by desmin. Immunolabelling of myosin isoforms can be used to assess the proportion of fast and slow fibres and there are usually only a few, or no, fibres containing fetal myosin in cases with mutations in the SELENON gene (previously known as SEPN1 ) (see below).
Clinical Features
A common phenotype associated with minicores shows marked axial weakness with spinal rigidity, scoliosis, torticollis and respiratory involvement that is often disproportionate to the overall muscle weakness. Patients are clinically similar to cases with the rigid spine syndrome and congenital muscular dystrophy (RSMD1), and the two disorders are considered allelic ( ). Both are caused by recessive mutations in the gene for selenoprotein N encoded by the gene now known as SELENON , and the spectrum also includes cases with Mallory bodies (Ferreiro et al 2004; see Chapter 12, Chapter 5 ).
Multiple minicores can also be seen in RYR1 -related myopathy with similar proximal and axial weakness and also showing partial or complete external ophthalmoplegia. Mutations (usually recessively inherited) in the RYR1 gene in these patients form part of the spectrum of changes associated with the RYR1 gene (see above; ), emphasizing the overlap in the core myopathies. Primary cardiac malfunction is not a typical feature of these groups of patients with multiple minicores, and many of the cases reported in the literature showing minicores with cardiac involvement are molecularly unsolved and probably heterogeneous. Some cases, however, have been shown to have a mutation in the MYH7 gene ( ) or the gene encoding titin ( ). Recently, two patients were identified with mutations in the ACTN2 gene encoding α-actinin 2 and biopsies showed unusual large multiple peripheral structured cores ( ); neither case, however, presented with hypotonia
Genotype–Phenotype Correlations
Recent studies have identified additional features that seem to be associated with minicores and a particular gene defect. In cases with SELENON mutations, a two-fibre type pattern is usually preserved and the minicores can occur in both fibre types (see Fig. 15.9 ), although type 1 fibres can be predominant. Other myopathic features include variation in fibre size, occasional, or at times, profuse internal nuclei, mild endomysial fibrosis and fat. The SELENON gene product is more abundant in fetal muscle, and this raises the possibility that muscle repair or regeneration may be influenced by the mutations, and some studies support this ( ). There are no studies of the localization of the protein in human muscle.
Cases with minicores associated with RYR1 mutations often have the clinical features described in central core disease (see above): in particular, central nuclei and type 1 fibre uniformity or predominance. The pathological features must always be considered, together with the clinical phenotype.
Prior to the molecular era, the muscle biopsies of several cases were reported with the presence of minicores in association with additional structural defects such as rods or whorled fibres ( ). Some have been shown to result from mutations in the RYR1 gene ( ) or the ACTA1 gene ( ), but many are molecularly unresolved.
Nemaline Myopathies
Nemaline myopathies are a clinically and genetically heterogenous group of myopathies (see table 15.2 ).
In described ‘nemaline myopathy, a new congenital myopathy’ in a 4-year-old girl who had been a floppy infant and had muscle weakness affecting the upper limbs more than the lower. As they were uncertain whether the rod-like structures in the muscle were separate rods or possibly an undulating thread-like structure, they suggested the name nemaline myopathy (Greek nema : thread). Also in independently observed ‘myogranules’ in the biopsy of a 4-year-old boy with hypotonia and non-progressive muscle weakness. The first description, however, is probably that of Reye in 1958 (see ), but the significance of it was uncertain at that time. There have been several subsequent reports of cases with rod-like structures with a wide range of phenotypes and several associated gene defects. The rods may occur in clusters, and always stain red with the Gomori trichrome technique.
There is a wide clinical spectrum associated with the presence of rods, even within families with the same mutation(s), ranging from severe neonatal forms to mild childhood-onset forms. Severely affected patients present with pronounced hypotonia and absence of both spontaneous movements and respiration at birth. Some are of antenatal onset within the spectrum of the fetal akinesia sequence ( ). Patients with an intermediate congenital form have antigravity movement and independent respiration at birth, but ambulation is not achieved and respiratory support is subsequently required. There is no particular genotype associated with this ‘intermediate’ form and it has been suggested that the clinical classification of nemaline myopathies proposed at the ENMC workshop in 1999 is updated ( ). A common form presents with hypotonia in early infancy or childhood, and patients have delayed motor milestones and generalized weakness predominantly affecting facial and axial muscles. They have a low muscle bulk, and feeding and respiratory problems are common. Independent ambulation in these cases is achieved, and the disorder is regarded as relatively non-progressive, or mildly progressive. Cases of adult onset have also been reported, including those referred to as sporadic late-onset nemaline myopathy (SLONM), many of whom are of autoimmune origin, or associated with HIV ( ). The condition in these patients is probably better described as a ‘myopathy with rods’ to distinguish it from childhood-onset nemaline myopathies with an underlying genetic cause. Some patients, however, with a defect in a gene that causes nemaline myopathy may not present until adulthood, but careful enquiry and clinical examination often identify problems that started in childhood, albeit mild.
Although most patients present with hypotonia, there are rare patients with hypertonia and stiffness ( ). Muscle weakness is usually generalized, with involvement of the neck flexors, the face and proximal muscles, often with a later, additional distal involvement. Distal weakness is a particular feature in some cases with mutations in NEB , but rods may not always be a feature in the muscle biopsies ( ) . Weakness of respiratory muscles is very common and an important clinical feature to monitor and manage ( ). Extraocular muscles are usually spared, except in patients with mutations in KLHL40 and LMOD3 , who frequently have ophthalmoplegia. Many of the reported patients with KLHL40 , KLHL41 and LMOD3 mutations have a severe phenotype but those with the apparent founder German/Austrian mutation have been reported to have a mild phenotype and survive into adulthood ( ).
Cardiomyopathy has been documented in some patients with defects in various ‘nemaline’ genes but is not usually considered a typical feature of nemaline myopathies ( ).
Histopathology
The defining feature of all nemaline myopathies, regardless of the gene defect, is the red-staining rods seen with the Gomori trichrome stain ( Fig. 15.10 ). In very young cases with very small fibres, they may be difficult to observe, and oil immersion optics of resin sections stained with toluidine blue (see Fig. 1.11 a), or electron microscopy, may be required. The rods are often clustered at the periphery of fibres, sometimes near nuclei, but can also be seen within fibres, sometimes in lines (see Fig. 15.10 and Fig. 11a ). In a few rare cases, rods are restricted to nuclei; in others they occur in nuclei and the cytoplasm (see Ch. 5 ). In most cases rods are only seen in the cytoplasm. The number of affected fibres varies between muscles, and the number of rods per fibre is also variable. There is no correlation between the number of rods and clinical severity. The presence of rods may be accompanied by accumulation of actin filaments (see below).

A wide variation in fibre size may be present, particularly in severe neonatal cases, when the fibres are generally small, but in childhood cases it is often less striking. Type 1 fibre atrophy (or type 1 hypotrophy when the fibres have never attained normal dimensions) and/or type 1 fibre uniformity or predominance is common. Type 2 fibres with fast myosin may be also be affected and be predominant ( Fig. 15.11a, b ). We have observed this is in biopsies from a few cases with NEB mutations (unpublished observation), and it has been reported in a case with a mutation in ACTA1 ( ). The type 1 fibre atrophy/hypotrophy and the restriction of rods to type 1 fibres in the rare cases with a mutation in the gene for α-tropomyosin probably relate to the restriction of the protein to type 1 fibres, but type 1 atrophy also occurs when other genes associated with nemaline myopathy are mutated ( Fig. 15.11c ). The involvement of type 2/fast fibres in patients with TNNT3 mutations relates to the expression of this troponin isoform in this fibre type ( ). Fibre hypertrophy may also be apparent (see Fig. 15.10c ). Internal nuclei, necrosis and fibrosis are not usually seen but can occur, for example, in patients with TNNT1 mutations ( Fig. 15.12 ). Similarly, regeneration is not a feature, although a few very small fibres with fetal myosin (‘pinpricks’) may be scattered throughout the biopsy, suggesting possible attempts at regeneration, but their origin is not known (see Fig. 6.25 ). Immature fibres with fetal myosin are often abundant in neonatal cases, and fast and slow myosin isoforms may be co-expressed in some fibres. Similarly, some immature fibres in neonatal cases may express the cardiac actin isoform, although in normal muscle this is usually down-regulated by birth ( ). In patients with 2 null mutations in the ACTA1 gene show that all muscle fibres express the cardiac actin isoform (see below). In two brothers with homozygous missense mutations in the ACTA1 gene, cardiac actin was more abundant than in control muscle but it was not detected in all fibres ( ). Biopsies from these rare cases also showed abundant cores, internal nuclei, split fibres and pathological features described as ‘dystrophic’, and electron microscopy revealed only a few rods.


Core-like areas and nemaline rods may occur in the same biopsy, again indicating the non-specificity of these structures and pathological overlap (see Fig 15.11c, d ; ). Areas with abundant rods lack mitochondria, and thus oxidative enzyme stains, and should be distinguished from the type of core-like areas described above that have myofibrillar disruption associated with an absence of mitochondria. The presence of more than one type of structural change rarely indicates a separate disease entity but emphasizes the histopathological overlap within the congenital myopathies and the spectra of abnormalities that can be associated with one gene (see Fig. 15.12 ).
With electron microscopy, rods are seen as electron-dense structures whose shape may be rod-like or sometimes ovoid, particularly in very young cases. Rods are often parallel to the longitudinal axis of the sarcomeres, and the appearance of their shape may be dependent on the plane of section ( Fig. 15.13a ). Rods may show continuity with Z lines, and irregularity of Z lines may also be apparent in some sarcomeres (see Fig. 15.13a ). In patients with mutations in the KLHL40 and LMOD3 genes, the rods in some fibres have a distinctive rectangular shape or resemble thickened Z lines ( Fig. 15.13b, c ), and the protruding myofibrils may have a fringe-like appearance. These unusual-shaped rods may occur in pairs in some cases with LMOD3 mutations ( Fig. 15.13d ; ). Cytoplasmic rods are considered to be derived from Z lines, as they show continuity with them, have a similar lattice structure and contain similar proteins. The major constituent of both rods and Z lines is α-actinin, but a number of other proteins have been demonstrated in them, including actin, tropomyosin, myotilin, γ-filamin, cofilin 2, telethonin and nebulin ( Fig. 15.14 ). Phalloidin binding highlights the presence of actin in rods (see Fig. 15.14c ). As with Z lines, desmin occurs at the periphery of rods but not within them. Current data suggest that nuclear rods, like cytoplasmic rods, contain α-actinin and actin, but in vitro studies suggest some differences between nuclear and cytoplasmic rods ( ).


Accumulation of actin thin filaments, with or without rods, or in association with various Z line abnormalities, is seen as pale staining areas with haematoxylin and eosin (H&E) and with the Gomori trichrome stain ( Fig. 15.15 ). It is a rare occurrence but has been observed in association with rods and mutations in ACTA1 and CFL2 ( ).

Various isoforms of α-actinin, a major component of rods, have been identified. The isoform encoded by the ACTN2 gene is present in all fibres but ACTN3 is restricted to a subset of type 2 fibres ( ). There is a homozygous polymorphism that is common in humans which obliterates the ACTN3 protein and seems to be associated with athletic performance ( ). Rods, in general, have the same isoform as the myofibrils. Most cases of nemaline myopathy have very few type 2 fibres and only ACTN2 is present but, in a few fibres, rods have been seen to label with antibodies to ACTN3 in addition to the ACTN2 of the myofibrils. These rods, therefore, have a different isoform from the myofibrils. The reason for this is not known but it may suggest that the rods are very stable structures. Mutations in the ACTN2 gene do not result in the formation of rods, but Z lines are disrupted and irregular, and oxidative enzyme stains reveal multiple cores at the periphery of fibres ( ).
Rod-like structures are not specific to the nemaline myopathies, and they can also be found at normal myotendinous junctions (see Fig. 3.8b ), in normal ocular muscles, in ageing muscle and occasionally in a variety of inherited and acquired neuromuscular disorders, such as sporadic late-onset nemaline myopathy (SLONM). As mentioned above, in some cases with a mutation in the RYR1 gene they may be a particular feature and be associated with cores, but other patients with RYR1 mutations may only show rods in a few fibres. Rods and cores have also been observed in association with defects in the genes encoding skeletal α-actin, nebulin and cofilin 2 TNNT1 and the KBTBD13 gene ( ). Confusion regarding disease entities can arise with overlapping pathology, and it is important to remember that not all cases with rods are necessarily congenital cases of ‘nemaline myopathy’, particularly adult cases, and, as discussed above, are better thought of as ‘myopathy with rods’. In biopsies described as ‘rod-core myopathy’ the rods and cores are present in separate fibres, suggesting that they are part of a spectrum of Z line abnormalities.
Cap-like areas are also associated with the genes associated with nemaline rod formation and have been linked with a disease entity (see below). They can also be considered to be part of the spectrum of myofibrillar and Z line abnormalities seen in nemaline myopathies, as both rods and caps can be seen in the same biopsy ( ) and in one family with a mutation in the TPM 2 gene the biopsy from one member showed rods while that from the other caps ( ).
Molecular Genetics
Nemaline myopathy can be inherited as an autosomal recessive or autosomal dominant disorder. There are also a number of de novo dominant cases. Mutations in at least 12 genes have been found to be associated with an abundance of rods. The defective genes encode thin filament proteins or proteins associated with thin filament regulation (see Table 15.1 ; ; ). Another gene, MYO18B, has also been reported in a single case, but it is not yet clear if this can be classified as a ‘nemaline myopathy’ as the patient had a complex phenotype that included dysmorphic features and cardiomyopathy ( ). Another publication reported two patients from different consanguineous families with MYO18B mutations and Klippel–Feil anomaly. Rods were observed in a muscle biopsy from one of these patients ( ). There is further genetic heterogeneity, as some cases do not link to any of the known loci and no pathogenic genetic defects have yet been identified. Additional genes are also associated with the presence of nemaline rods or cap-like areas, but they are not usually defined as ‘nemaline myopathy’, although publications may refer to them as such. For example, RYR1 and TTN (see below) , encoding the ryanodine receptor 1 and titin, respectively ( ), EXOSC3 that encodes a component of the human RNA exosome complex, RYR3 encoding the ryanodine receptor 3 ( ); one patient with mutations in the PPA2 gene that encodes the mitochondrial pyrophosphatase and causes sudden cardiac death was reported to show nemaline rods in skeletal muscle ( ).
Mutations in the ACTA1 and NEB genes are currently the most common causes of nemaline myopathy. Mutations in ACTA1 are mainly dominantly inherited or de novo dominant with a few rare recessive cases ( ). In contrast, mutations in the gene for nebulin, NEB, are recessively inherited with the exception of one dominantly inherited mutation in a patient with a distal myopathy ( , ). Many mutations are now identified by screening a panel of known genes associated with nemaline myopathy, but there is often difficulty in screening the 183 exons of the large NEB gene. The gene has multiple splice sites and a triplicate repeat region, where the most common large variants of the gene are found, and the gene gives rise to many isoforms in both skeletal muscle and brain ( ) . Identifying both mutations in NEB in a patient may be difficult, and determining the pathogenicity of especially missense variants constitutes a further diagnostic challenge. Recent advances include a targeted array which, in many patients, has helped to identify the second mutation ( ), and also the development of functional assays for testing the effects on the protein of missense variants ( ).
Nemaline myopathies geographically occur all over the world, but some mutations have arisen as founder mutations and are thus prevalent in particular populations. For example, the deletion of the entire exon 55 of NEB occurs in people of Ashkenazi Jewish ancestry, who have now populated many parts of the world ( ). Probable founder mutations have also been identified in TNNT1 ( E180X in exon 11 causing a stop codon) in the Amish population ( ), TPM3 (deletion of the first nucleotide of the last exon, c.913delA) in the Turkish population ( ), KLHL40 (c.1582G>A) in the Japanese, Kurdish and Turkish populations ( ), ACTA1 ( p.Asp181fsX10) in the Pakistani population ( ), LMOD3 (c.1648c>T) in German and Austrian populations ( ), and three mutations in NEB (p.Ser6366Ile in ex122, p.Thr7382Pro in ex151 and p.Thr6350Profs∗4 in ex122) in the Finnish population ( ). Although mutations in TNNT1 encoding troponin T were first identified in the Amish population, a few patients of Dutch descent, and others of non-Amish origin have also been described ( ).
Mutations in the TPM2 and TPM3 genes are associated with other phenotypes and pathologies (see below) and again illustrate the overlap.
Genotype–Phenotype Correlations
It is rarely possible to predict the defective gene responsible for a nemaline myopathy from the pathology alone. Mutations in the genes for actin and nebulin are the most common and the pathology must be considered with the clinical phenotype. Several severe neonatal patients with nemaline myopathy have a mutation in the ACTA1 gene, but some severely affected patients with NEB mutations have also been described ( ). In addition, several of the reported cases with mutations in the rarely affected genes have homozygous mutations and are severely affected: e.g. CFL2, KLHL40 and LMOD3. The Austrian/German patients with a possible founder mutation in LMOD3 , however, have a milder phenotype ( ). Muscle MRI is also proving to be helpful in relation to specific patterns of muscle involvement ( ). The presence of nuclear rods and/or accumulation of actin thin filaments was considered to be useful for predicting an ACTA1 mutation, but these have also been observed in the rare cases of nemaline myopathy caused by mutations in CFL2 (actin accumulation) and MYPN (nuclear rods). Nuclear rods have also been observed in cases with mutations encoding plectin or ZASP, but their phenotype is not that of a congenital myopathy ( ). Expression of the cardiac actin isoform in all fibres has only been observed in cases homozygous for null mutations for ACTA1 ( ). These cases illustrate that the absence of skeletal actin is not lethal and that it is not required for the formation of rods.
The ACTA1 gene is also responsible for the disorder named zebra body myopathy ( ), and this disorder is thus better considered as part of the nemaline, ACTA1 spectrum. Only two cases have been reported in the literature ( ), and both not only showed zebra bodies but also nemaline rods. Re-evaluation of a second biopsy from the original case of ‘zebra body myopathy’ performed at the age of 29 years revealed a variety of pathological features, including accumulation of actin filaments and nuclear rods. Ring-like arrangement of myofibrils was also observed in this patient, which is also a feature of some animal models of ACTA1 ( ). This rare case of ‘zebra body myopathy’ illustrates that both accumulation of actin and nuclear rods can occur in more mildly affected cases as well as severely affected ones.
Immunolabelling with commercial antibodies to nebulin usually reveals the presence of the protein, although many of the mutations result in a truncated protein. Research studies using a non-commercial antibody to the SH3 domain of nebulin suggested that its absence could help identify a mutation in the nebulin gene in severe cases ( ). Subsequently, however, secondary changes in nebulin have also been identified in severe ACTA1 cases ( ). In addition, electron microscopy studies have suggested that cases with nebulin mutations show an indistinct H zone of the myofibrils, but the specificity of this finding is uncertain as the H zone is often indistinct when the myofibrils are contracted ( ). Monoclonal antibodies that specifically recognize the differentially sliced 143 and 144 exons of nebulin indicate development regulation and that exon 144 is present early in myogenesis but not exon 143. Mature muscle fibres with fast myosin express exon 143 (see Fig 6.14 ; ).
Myotubular/Centronuclear Myopathies
In 1966, Spiro and co-workers suggested the name ‘myotubular myopathy’ for the histological changes observed in the biopsies from a 12-year-old boy, because of the striking resemblance to the myotubes of fetal muscle. On biopsy of the gastrocnemius, the muscle fibres were normal in size, but in about 85% of fibres there were one to four centrally or internally placed nuclei, surrounded by an area devoid of myofibrils. Similar changes were noted in about 45% of fibres in a second biopsy. Histochemical studies showed normal oxidative enzyme activity in unaffected fibres, but in affected fibres there was a central zone either devoid of enzyme activity or with increased enzyme activity. Phosphorylase and PAS activity also showed either an increase or a decrease in the central zone of respective fibres. The ATPase reaction, on the other hand, showed a consistent absence of activity in these central areas. On electron microscopy, the perinuclear zone contained aggregates of mitochondria and myelin figures but there were no ribosomal aggregates. considered these abnormal fibres to be comparable to the myotubes of developing muscle and postulated an arrest in the development of the muscle at a cellular level.
Subsequently, observed similar pathological changes in the muscle of two African sisters, aged 18 and 16 years, and their symptom-free mother. The first sister had delay in early motor milestones, generalized atrophy of the musculature, a slowly progressive weakness of the skeletal muscles, ptosis, external ophthalmoplegia and facial weakness. The clinical photograph with the long, thin face showed a striking resemblance to the case of and also to the ‘dysmorphic’ features of some reported cases of nemaline myopathy. The younger sister, who had not been floppy as an infant and had no delay in motor milestones, showed a diffuse wasting and weakness of the limbs and bilateral ptosis, but no facial weakness or ophthalmoplegia.
Biopsy of the rectus femoris and gastrocnemius in both sisters showed central nuclei in the majority of fibres. In the mother’s gastrocnemius, about a third of the fibres had internal nuclei. Fibre diameter was normal in all three, with histochemical differentiation of type 1 and 2 fibres. Oxidative enzyme activity was either concentrated or absent in the central zones of the fibres with central nuclei. Phosphorylase and glycogen showed an intracellular distribution similar to the dehydrogenases. Myofibrillar ATPase was lacking in the central zones. Electron microscopy confirmed the presence of central nuclei and showed other non-specific changes in the central region of the fibres. were not convinced of the pathogenesis of the condition and suggested the alternative descriptive title ‘centronuclear myopathy’ rather than ‘myotubular’. The term ‘myotubular’ is firmly entrenched in the literature and will probably persist in spite of the possibly erroneous premise for its choice. Since these early descriptions, many cases with prominent central nuclei associated with various genetic defects have been identified. They are clinically heterogeneous with neonatal, childhood or adult onset and an X-linked or autosomal inheritance identified. The X-linked form usually has a severe neonatal presentation and the term ‘myotubular’ is now often restricted to these cases, while the more descriptive term of ‘centronuclear’ is applied to the autosomal cases. The historical cases described above were not molecularly resolved and with current knowledge of the clinical and pathological spectra in the congenital myopathies they may have been cases of ‘centronuclear-RYR1’ (see below).
X-linked Myotubular Myopathy
This is a severe condition with onset in utero. Pregnancy is complicated by polyhydramnios, and there is often a history of miscarriages and neonatal death in the maternal line. There is marked neonatal hypotonia, a variable degree of external ophthalmoplegia, feeding difficulties and respiratory failure at birth, which is often fatal. Some severely affected infants may survive if the respiratory problems in the neonatal period can be overcome ( ). The molecular defect is a mutation in the MTM1 gene on the X chromosome, encoding a protein named myotubularin (see below).
Histopathology
The key feature is the large centrally placed nuclei ( Fig. 15.16a ). In longitudinal section, these are regularly spaced down the fibre ( Fig. 15.16b ), in contrast to the chains of central nuclei in regenerating fibres; thus, the plane of section influences the number of central nuclei observed in transverse section. The number of central nuclei can vary between muscles, and they may not always be numerous or apparent at birth ( , ). Central nuclei occur in both fibre types.
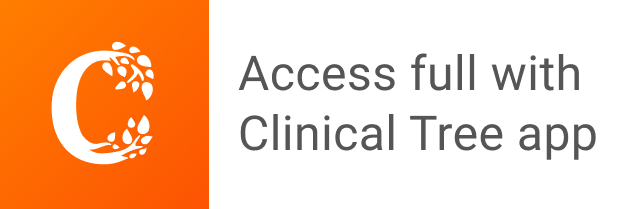