Introduction
Biomechanics is the study of engineering mechanics, specifically Newton’s laws, as applied to the musculoskeletal system. An understanding of the biomechanics of the foot and ankle enables appreciation of the intricate function of individual components, their inter-relationships, and aids in an understanding of pathology and the potential impact of surgical intervention. Although the focus of this chapter is on foot and ankle biomechanics, the foot and ankle should not be considered in isolation, but as an open chain. Foot and ankle biomechanics are intimately related to the knee, hip, and spine. The principles are outlined with clinical correlates.
Function
The foot has evolved for bipedal locomotion, providing a mechanism for the absorption and transfer of energy during propulsion, and stability during stance. Additionally, the foot is important for balance and provides an interface with the environment.
Energy Absorption and Transfer
The foot has a unique ability to change from a flexible, compliant structure to a rigid lever. To absorb and transfer energy efficiently it must be able to conform to even and uneven surfaces and make good contact with almost any supporting surface, while also forming a rigid platform that does not collapse under body weight. The forces transmitted through the foot during walking and running are between one and three times body weight1 and up to 16 times in jumping2. Energy is either dissipated or absorbed by the foot, protecting the skeletal structures more proximally. The specialized cells of the plantar skin, subcutaneous fat pads under the heel and metatarsal heads, plantar aponeurosis, ligaments, and the intrinsic and extrinsic musculature all absorb the forces and store the potential energy. The plantar aponeurosis and musculature release the energy during propulsive activity.
Stability
A combination of dynamic and static stabilizers created by the relatively stiff medial longitudinal arch, bony congruence, the close packing of individual bones in certain foot positions, the plantar soft tissue structures, and traversing musculature maintain foot stability during standing, walking, or running.
Proprioceptive nerve endings within the intrinsic musculature, ligaments, and joint capsules feed back to allow the intrinsic and extrinsic musculature to maintain the center of gravity in a 4 cm2 area just anterior to the ankle joint3. In the resting state, soleus, tibialis posterior, and peroneus brevis control anteroposterior oscillations4. In patients who have been immobilized in cast, the proprioceptive mechanism becomes dormant, making balance difficult when standing and walking unsteady.
Sensory
The skin in contact with the ground can be over 5 mm thick in unshod individuals5. It responds to increases in pressure by creating callosities to reduce soft tissue trauma. The specialized skin covering the surface of the foot is also densely populated with nociceptive fibers, which sense pain and temperature, enabling subtle and constant changes in pressure by constantly adjusting the center of gravity. Neuropathic individuals, for example diabetics, lose the ability to sense increases in pressure or trauma to the foot, resulting in ulceration. Neuropathy also affects proprioception and thus balance.
Evolution of the Foot
A detailed treatise on the evolution of the foot is beyond the scope of this chapter but, for the interested reader, Olson and Seidel (1983)6 published an in-depth review. There are a number of key developments worth highlighting. The origins of the foot are from tree-dwelling apes in which the “foot” functioned as a grasping appendage (Figure 2.1). Although the foot has been evolving for over 2 million years, the anatomical variation found within the human population suggests that the foot remains a work in progress7. The foot is evolving to improve its function in balance, propulsion, and upright stance8.
Figure 2.1 The major evolutionary changes in foot morphology including a broader and straighter first ray, longer calcaneus, and higher medial arch. The human left foot (top and left), and simian left foot (lower) and right foot (right).
While the lateral torsion in the first ray and slight medial torsion in the lateral four rays are features of a foot once used for grasping, many other features reflect its adaption for weight bearing. The human calcaneus is larger than its primate origins, resulting in a longer moment arm for the tendo Achillis to improve propulsion. The plantar surface is also larger, reducing point loading by increasing the contact area. The subtalar facet joints are less obliquely oriented relative to the ground, reducing the shear stresses through the joint in bipedal locomotion.
The bony architecture of the midfoot has also changed. The cuboid and cuneiforms have evolved to become wedge shaped, apex plantarwards, in the formation of the transverse arch. This has been further strengthened by the relative hypertrophy of the plantar ligaments. The overall effect is to make the midfoot more rigid, with the ability to absorb and store energy during standing and locomotion.
The first ray is broader and straighter than the other four rays with less transverse and sagittal plane motion, and is almost parallel with the second ray. Stability is also conferred by slightly flatter first metatarsocuneiform joint and the surrounding intrinsic musculature and deep transverse metatarsal ligament, which is unique to humans.
A recent study by Wang et al. (2014)10 compared foot structure in humans and apes, examining the influence of foot proportions on force, torque, and work in the foot joints during simulated bipedal walking. The authors concluded that the geometric proportions of the feet in humans and apes exert a discernible effect on these variables. Their results showed that during simulated human-like bipedal walking: (1) the human and ape feet experience similar joint forces, although the distributions of the forces differ; (2) the ape foot incurs larger torque across the joint than the human foot. The human foot has higher torque in the first tarsometatarsal and metatarsophalangeal joints, whereas the ape foot incurs higher torque in the lateral digits; (3) total work in the metatarsophalangeal joints is lower in the human foot than in the ape foot.
Thus the proportions of the human foot are more advantageous for bipedal walking than the ape’s. The authors have also shown that short toes play an important role in human walking. These subtle changes have improved the propulsive potential of the foot as well as its stability, and created an important evolutionary step, freeing the upper limb to develop fine motor skills with parallel brain development.
Anatomy
The soft tissue structures are built around and on a constrained bony anatomy comprising 26 bones, which, for practical purposes, are grouped into the hindfoot, midfoot, and forefoot (Figure 2.2a).
(a) The 26 bones of the foot and their division into hindfoot, midfoot, and forefoot.
(b) Interosseous trabecular patterns of the ankle and foot.
The hindfoot comprises the talus and calcaneus, which are the first and second bones to ossify, respectively. The three parts of the talus (body, neck, and head) are oriented to transmit reactive forces from the foot through the ankle to the leg. Lying between the calcaneus and tibia, the talus communicates thrust from one to the other. The calcaneus is the largest and most posterior bone in the foot and provides a lever arm for the insertion of the tendo Achillis and associated triceps surae, which imparts plantar flexion forces to the foot. The calcaneus’ height, width, and structure enable it to withstand high tensile, bending and compressive forces. Trabecular lines, formed according to Wolff’s law, run through the hindfoot. They are contiguous with the trabecular patterns from the tibia and extend into the midfoot and forefoot, crossing joints. The anterior trabecular system in the tibia curves posteriorly into the talus and posterior calcaneus. The posterior trabecular system in the tibia curves anteriorly extending into the medial column. A plantar-based system joins the posterior and anterior systems and is accompanied by a number of smaller systems within each of the bones of the foot (Figure 2.2b).
The midfoot comprises the navicular, cuboid, and the three cuneiforms – medial, intermediate, and lateral. The navicular is boat shaped, as its name suggests, and articulates with the head of the talus proximally and the three cuneiforms distally. The navicular forms the keystone at the apex of the medial longitudinal arch. It is the last bone to ossify in the foot. These features may be why it is vulnerable to osteochondrosis (Köhler’s disease) in childhood and vulnerable to the development of avascular necrosis11. The cuboid articulates with the calcaneus proximally, the lateral cuneiform medially, and the fourth and fifth metatarsals distally. In a small proportion of the population, there is an articulation between the navicular and the cuboid, and occasionally the two are joined as a coalition12. The three cuneiforms are trapezoidal, with a broad dorsal aspect and narrower plantar surface. They form an intrinsically stable transverse arch. The medial, intermediate, and lateral cuneiforms articulate individually with the first, second, and third metatarsals in turn.
The forefoot comprises the metatarsals and phalanges. There are five metatarsals in the forefoot. These are all tapered distally and articulate with the proximal phalanges. The first metatarsal is the shortest and widest. The head of the first metatarsal also articulates with two sesamoids, the tibial and fibular sesamoids, which are situated within the flexor hallucis brevis tendon on the plantar articular surface. The second metatarsal is recessed proximally between the medial and lateral cuneiforms, and articulates with the intermediate cuneiform. This results in the second metatarsal being “locked” in place. The third, fourth, and fifth metatarsals are broad at the base and narrow in the shaft. The fifth has a prominent styloid, laterally and proximally at its base, to which the peroneus brevis tendon and plantar fascia are attached. The phalanges represent the digits. The big toe (hallux) consists of two phalanges and the remaining four toes typically have three phalanges (Figures 2.2a). The heads of the proximal and middle phalanges tend to be trochlear shaped, creating good stability.
Joint Motion
By convention, motion occurs around three axes: X, Y, and Z (Figure 2.3). Plantar flexion and dorsiflexion describe sagittal plane motion (Y–Z plane), inversion and eversion describe coronal plane motion (X–Y), and adduction and abduction describe transverse plane motion (Z–X).
Figure 2.3 Convention for the axes around the foot and the associated motion around each axis.
The foot needs to remain flat on the ground and must accommodate the rotational moments that are transmitted from the pelvis and lower limb to enable efficient forward propulsion during gait. This is accomplished through composite motion created by the variable axes of each of the joints of the foot and ankle. There is a marked degree of variability in the range of motion of each joint within the normal population. This variability can result in variation of foot posture, which, in turn, defines an individual’s gait.
Pronation and supination are composite triplanar motions of the foot: pronation describes abduction in the transverse plane, dorsiflexion in the sagittal plane, and eversion in the coronal plane; supination is the opposite, i.e., adduction, plantar flexion, and inversion.
The next section covers each of the major joints in turn. For several reasons, considering a joint axis as a simple hinge joint is an oversimplification. Firstly, arbitrarily selecting sagittal, coronal, and transverse planes for reference means that out-of-plane joints will, by definition, produce multiplanar motion. Secondly, the varying topography and congruency between articular surfaces results in an instantaneously changing center of rotation during motion. The result is motion around a series of helical axes, exemplified at the ankle and subtalar joints12.
Ankle Joint
Inman describes the ankle joint as uniaxial, with its axis a few millimeters distal to the tips of the malleoli and a few millimeters anterior to the lateral malleolus13. The result is a downward and laterally oriented axis with a range in the coronal plane from 74 to 94° (Figure 2.4).
Figure 2.4 Orientation of the right ankle joint axis (frontal view).
There is great variability in the ankle joint range of motion described in the literature. This probably reflects the differences in methodology. For example, Sammarco et al. (1973)14 reported 23 ± 7° of ankle dorsiflexion and 23 ± 8° of plantar flexion under weightbearing conditions. However, only 10° of dorsiflexion and 20° of plantar flexion are deemed necessary for normal locomotion15.
Additionally, there is variation in the curvature of the lateral and medial components of the talar dome, which results in differing axes of rotation in plantar flexion and dorsiflexion16; this accounts for the foot being 1° internally rotated in plantar flexion and 9° externally rotated in dorsiflexion17 (Figure 2.5).
Figure 2.5 Toe-in and toe-out foot motion.
There is also tibial rotation during the normal gait cycle. During the early stance phase, with forward progression of the tibia and ankle dorsiflexion, there is internal tibial rotation. In contrast, in late stance phase, with the ankle plantar flexing, there is external tibial rotation13.
The position of arthrodesis of the ankle joint in all three planes is critical to the function of the joints above and below the ankle. Fusion in plantar flexion results in an increase in leg length, with a back-knee thrust and increased stress across the midfoot. By contrast, fusion of the ankle in dorsiflexion results in excessive loading of the heel. Fusion with the ankle in internal rotation results in increased stresses at the knee and subtalar joint, and external rotation may increase loading at the first MTPJ and can lead to the development of hallux valgus. Finally, varus positioning of the talus can lead to lateral column loading as well as increased stress through the midfoot, if subtalar joint motion is limited18.
The ankle is stabilized by the congruent articular surfaces, the ligaments, and the muscles traversing joint. The congruency of the bony structures is particularly important during weight bearing and is dependent upon the position of the talus within the mortise, being less stable in extreme plantar flexion.
The ligamentous structures around the ankle joint are critical in the unloaded ankle, with each ligament providing variable contributions depending on the position of the foot and ankle. Serial sectioning reveals the relative contributions of each ligament and their primary mode of failure19: the anterior talofibular ligament (ATFL) is the most commonly injured ligament and primarily prevents anterior translation of the talus, resists internal rotation of the talus in the ankle mortise, and resists adduction when the foot is plantar flexed; the calcaneofibular ligament (CFL) also resists adduction but its primary role is with the foot in neutral or dorsiflexed; the posterior talofibular ligament (PTFL) and deep deltoid resist external rotation of the dorsiflexed ankle. Finally, extreme dorsiflexion is resisted by the posterior tibiotalar ligament.
Peroneus longus and peroneus brevis muscle activity further protects against lateral ankle ligament injury when subjected to external forces20–21. Additionally, tibialis anterior, extensor digitorum longus, extensor digitorum brevis, and peroneus tertius play a role through eccentric contraction – resisting forced supination of the hindfoot and protecting the lateral ligament structures.
The Subtalar Joint
The subtalar joint is the articulation between the talus and calcaneus, which has three facets – posterior, middle, and anterior. Like the ankle joint, it is a single axis joint, which is angled 16° medial to the longitudinal axis of the foot and slopes 42° inferolaterally compared to the transverse plane (Figure 2.6)22. Nevertheless, there is a wide degree of variation.
Figure 2.6 The orientation of the subtalar joint axis in (a) the sagittal plane; (b) the transverse plane.
There are two functional components: the posterior talocalcaneal facet, and the talocalcaneonavicular joint or “acetabulum pedis.” The acetabulum pedis is a deep socket that accommodates the head of the talus. It is made up of the navicular, the anterior and middle facets of the calcaneus, and the spring ligament complex (superomedial and inferior calcaneonavicular ligaments) (Figure 2.7).
Figure 2.7 Components of the acetabulum pedis. View with the talar head removed.
The oval shape of the articular surfaces and oblique orientation of the subtalar joint axis means that the subtalar joint allows the coupled movements of supination and pronation; it also enables the joint to act as a shock absorber and torque converter, converting rotation around a vertical axis to rotation around a horizontal axis, while minimizing shear through adjacent joints as energy is transferred; the ankle and subtalar joints, considered together, accommodate 19° of transverse plane rotation of the tibia, 8° through the subtalar joint and 11° through the ankle. As described above, in early stance phase, as the tibia shifts forward over the dorsiflexing ankle it rotates internally and, at the same time, the calcaneus everts. In contrast, during late stance phase, as the ankle plantar flexes, the tibia externally rotates and the calcaneus inverts. These small movements are vital for the foot to accommodate uneven surfaces.
Tarsal coalition is a congenital anomaly, which can cause a painful flat foot. Calcaneonavicular or talocalcaneal coalition reduces subtalar movement and increases stress across the proximal and distal joints. The loss of the torque converter function of the subtalar joint may be taken up by the ankle joint, which, in the developing skeleton, can result in the development of a ball-and-socket ankle joint. Furthermore, the gliding motion is also restricted and the joints act as a hinge, with periosteal damage and the talar beaking that is a radiographic feature of this condition23.
The subtalar joint is stabilized by components of the deltoid and lateral collateral ligaments, the interosseous ligaments of the sinus tarsi, and by its lateral capsule12. The ligaments of the sinus tarsi separate the posterior facet from the anterior and middle facets of the subtalar joint. The interosseous talocalcaneal ligament within the tarsal canal is the most important as it limits eversion. It consists of two thick short bands, which act as the center of rotation for the subtalar joint. Additionally, there are four weaker ligaments, which contribute to overall stability: the anterior talocalcaneal ligament; posterior talocalcaneal ligament; lateral talocalcaneal ligament; and calcaneofibular ligament. The tibialis posterior, flexor hallucis longus, and flexor digitorum longus provide dynamic stability to the hindfoot in general.
Transverse Tarsal Joint (Chopart’s Joint)
The rotation that occurs at the subtalar joint would translate along the whole of the forefoot, everting and inverting it with tibial rotation during gait. However, the transverse tarsal joint, or Chopart’s joint, allows movement and acts as a pivot in the midfoot. It consists of the talocalcaneonavicular and calcaneocuboid joints and is the key to enabling transition from a flexible to a rigid structure. The talocalcaneonavicular joint functions as a ball-and-socket joint in a similar way to the hip joint – hence the acetabulum pedis. The calcaneocuboid is saddle shaped. The convex and concave surfaces are perpendicular to each other; the convex surface is horizontal and the concave surface is vertical. The degree of congruency between the two surfaces means that motion is essentially limited to rotation.
The calcaneocuboid and talonavicular joints work together; they are parallel when the calcaneus is everted (Figure 2.8a) and non-parallel when the calcaneus is inverted (Figure 2.8b). Thus as the foot makes contact with the ground, the heel is everted, the joints are parallel, and hence the foot is flexible, accommodating any unevenness and absorbing the impact. As the calcaneus inverts, the joints diverge, converting the foot into a rigid lever upon which the tendo Achillis can act to push off24. In the parallel configuration, joint motion occurs around two axes: the longitudinal axis slopes medially and upward enabling inversion and eversion; the second oblique axis allows dorsiflexion/abduction and plantar flexion/adduction16. The composite motion (pronation/supination) results in a plantigrade foot with varus and valgus hindfoot motion.
Figure 2.8 Relative change in orientation of the calcaneocuboid and talonavicular joints converting the foot from a compliant to a stiff structure. A view of the midfoot, navicular and cuboid removed. Talar head and anterior calcaneus shaded. As the heel goes into varus (b) the joints diverge.
The importance of the talonavicular joint is reflected in the effect of fusion on the remaining hindfoot mechanics. Astion et al. (1997)25 found that fusion of the talonavicular joint, either alone or in combination with either the subtalar joint or calcaneocuboid joint, limited the motion of the remaining joints to 2°, effectively “locking” the triple complex.
The main support of the transverse tarsal joints is ligamentous. The four ligaments connecting the calcaneus and cuboid are the medial, dorsolateral, long, and short plantar ligaments. The medial calcaneocuboid ligament forms part of the bifurcate ligament, with the calcaneonavicular ligament, or ligament of Chopart. The anterior talonavicular capsule is defective, anteriorly, and is reinforced by the talonavicular ligament and the lateral calcaneonavicular ligament. Inferomedially the capsule is strengthened by the inferior calcaneonavicular ligament (the spring ligament)12. Further stability in subtalar inversion is provided by the two joints diverging, as described above.
Intertarsal Joints
The naviculocuboid, naviculocuneiform, and intercuneiform joints that form the arch of the midfoot are, individually, relatively rigid. However, their cumulative motion enables the transverse arch to flatten and rise with hindfoot pronation and supination, respectively. Stability is conferred by the bony congruence, and dorsal and plantar ligaments. The plantar ligaments are the strongest.
Tarsometatarsal Joints (Lisfranc)
The TMTJ consists of the three cuneiforms and their articulation with the first, second, and third metatarsals, as well as the cuboid articulation with the fourth and fifth metatarsals. In the coronal plane the tarsal bones are trapezoidal in shape, creating a “Roman arch.” The configuration is stable in compression. Stability of the medial column is partially bony, with the long second metatarsal locked between the bases of the first and third metatarsals and the three cuneiforms. The articular surfaces are relatively flat, limiting overall motion. There are also strong dorsal, plantar, and intermetatarsal ligaments, which stabilize the TMTJ. The relatively stronger plantar ligaments resist flattening of the arch during stance. However, the largest and strongest interosseous ligament is the Lisfranc ligament, which arises from the lateral surface of the medial cuneiform and inserts onto the medial aspect of the second metatarsal base near the plantar surface. The integrity of the Lisfranc ligament is considered crucial to the integrity of the TMTJ complex12. The first metatarsal base is anchored to the medial cuneiform by plantar and dorsal longitudinal ligaments. The peroneus longus attachment to the lateral base of the first metatarsal provides dynamic resistance to first metatarsal varus and supination of the forefoot during toe off. The tibialis anterior also stabilizes the first TMTJ. Longitudinal and oblique ligaments stabilize the remainder of the metatarsals to the cuneiforms and cuboid on the dorsal and plantar surfaces. The first to third TMTJs have little motion, with the second TMTJ being the stiffest of all.
By contrast, the fourth and fifth TMTJs are mobile with approximately twice the range of motion of the medial three rays. This relative difference reflects the functional demands, with the stiffer medial column allowing a more efficient transfer of energy and creating a more effective moment arm for the tendo Achillis. The flexible lateral column enables the foot to accommodate variation in terrain with shock absorption and equitable distribution of force through the midfoot.
The importance of lateral column mobility makes the management of symptomatic degenerative change of the fourth and fifth TMTJs challenging. Thus surgery for degenerative change of the TMTJs is focused on fusion of the first, second, and third TMTJs, with retention of mobility of the fourth and fifth TMTJs. The outcome for lateral column fusion is generally poor26, consequently replacement and interposition arthroplasty with tendon have been tried.
Forefoot
The forefoot comprises the metatarsals and phalanges with their associated joints. The metatarsophalangeal joints (MTPJs) form an arch in the transverse plane. Two distinctive foot types have been recognized in both science and in art: one with a long first ray, the “Greek” or “Roman” foot, and the other with a relatively long second ray, the “Egyptian” foot. The long first ray was recognized in Assyrian and Greek art and is later reflected in work by Versalius and Leonardo da Vinci after its adoption by the Romans. This contrasts with Egyptian, Mexican, and Nigerian art, which depicts the second ray as longer. Morton (1927)27 described a congenitally short first metatarsal as part of a triad, along with a hypermobile first ray and calluses under the second and third metatarsal heads, calling it metatarsus atavicus, implying atavism, or an evolutionary throwback.
The long second toe is thought to affect less than 10% of the population and is clinically more relevant in shod societies where the second toe can impinge on the toe box of the shoe. The relevance of relative toe length also relates to gait and the initial phase of push off, which occurs through an oblique axis, which passes through the second to fifth metatarsal heads – the oblique metatarsophalangeal break line (Figure 2.9). The angle of the break line varies between 50 and 70° in the transverse plane28, and enables the tibial axis to remain vertical at toe off with the majority of force transmitted through the medial column of the first three rays.
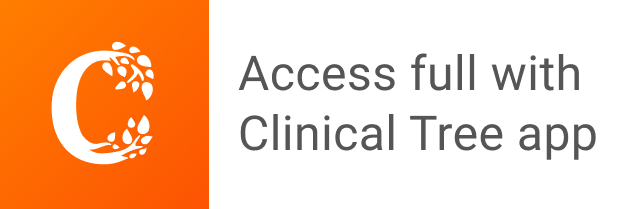