As awareness on the short-term and long-term consequences of sports-related concussions and repetitive head impacts continues to grow, so too does the necessity to establish biomechanical measures of risk that inform public policy and risk mitigation strategies. A more precise exposure metric is central to establishing relationships among the traumatic experience, risk, and ultimately clinical outcomes. Accurate exposure metrics provide a means to support evidence-informed decisions accelerating public policy mandating brain trauma management through sport modification and safer play.
Key points
- •
Improved metrics of cumulative exposure to repetitive head impacts are needed to draw relationships between trauma and risk of neurologic injury.
- •
Routine symptom-based assessments are not designed to capture the range of trauma experienced within sport defined by structural and functional changes within the brain.
- •
Brain trauma profiling is an objective measurement method of quantifying cumulative exposure by incorporating the characteristics that associate with risk to brain injury.
Introduction
As knowledge of short-term and long-term consequences of sports-related concussions (SRC) continues to grow, so too does the necessity of establishing measures of brain trauma that inform public policy and risk mitigation strategies. Cumulative exposure from repetitive head impacts (RHI) pose a variety of risks, including increased concussion susceptibility, mental health disorders, cognitive impairments, and brain disease pathologies, including chronic traumatic encephalopathy (CTE). Physical trauma to neurons disrupts a number of processes, many with acute and chronic consequences that may not express as immediately recognizable injury, , but rather develop over time. , Clinical definitions of concussion depend on immediate and transient expression, and recognition and documentation of signs and symptoms. Incidence estimates of SRC therefore account for recognized and reported symptoms; however, fail to account for underreporting and cumulative RHI and provide limited understanding of the injury itself defined by functional and structural cellular changes. As a result, relying on reported and incidence rates of SRC as an indicator of risk and injury is inconsistent, subjective, and only captures one level of trauma. As the appreciation of the complexity of the mechanisms and neurologic outcomes of traumatic brain injury (TBI) in sport is realized, there has come a broader recognition of the public health problem being faced. Large-scale questions remain regarding population-based and individual risks, dose-response relationships, and evidence-informed strategies for risk reduction.
This article describes the need to develop improved biomechanical metrics of brain trauma including cumulative exposure. Advances in understanding brain injury in sport continue as evidence reveals the inadequacy of using single-event metrics, acute clinical expression, or concussion incidence as measures of trauma. A review of the neurobiological underpinnings of neurotrauma is presented, described, and incorporated into redefining the biomechanics of neurologic injury beyond the current, rather ambiguous, definitions. An objective method of capturing and quantifying RHI exposure by identifying and integrating the biomechanical characteristics that associate with risk of neurologic injury is needed. A more precise measure of exposure is central to establishing relationships between the traumatic event(s), risk and clinical outcomes. Accurate exposure metrics provide a means to support evidence-informed decisions accelerating public policy mandating RHI management through sport modification and safer play.
Sports-related concussion
The challenge in establishing a universal consensus on SRC is complicated by the subjective nature of its definition. The result is that SRC largely remain underreported and undiagnosed, , estimated at as little as 1 in 6. In many cases, recognizing symptoms and reporting a concussion is primarily the responsibility of the athlete, including young athletes with limited knowledge and resources. Educational programs may have limited reach, as self-reporting requires the athlete not only to recognize the injury but disclose symptoms. Kroshus and colleagues demonstrated that educating National Collegiate Athletic Association (NCAA) hockey players was an ineffective intervention to increasing player safety, as minimal behavior change in voluntary reporting was observed. Young athletes face social and performance pressures that discourage reporting, such as fear of being removed from the game, letting down teammates and coaches, missing practice, and looking weak. , Challenges in recognizing, reporting, and diagnosing concussion, limits the use of concussion data as an accurate measure for trauma in sport. Acute symptoms following an impact often represent an individual response to trauma and do not account for asymptomatic expression or cumulative RHI. Cumulative RHI associates with microstructural changes within the brain and presents as changes in function, connectivity, activation, and cognition in athletes playing various sports in the absence of diagnosed injury. The consequence is that many athletes experience unrecognized RHI throughout the season, where they continue to participate seemingly unharmed. The most severe consequence of RHI is its association with CTE, progressive brain disease affecting the integrity of cell proteins. Incomplete reporting, ambiguous presentation, and poor definition of what is regarded as a tissue “injury” presents a challenge when defining relationships between trauma and risk. Establishing these relationships largely relies on how exposure is measured.
Biomechanics of traumatic brain injury
Mechanisms and Metrics of Head Injury
Biomechanics of head injury aim to understand the mechanical circumstances leading to the initial trauma and are the starting point to addressing global TBI pathophysiology. Injury mechanism describes the mechanical and physical changes that result in structural and/or functional damage. Biomechanical research on TBI is rooted in historic investigations using cadaver and animal models examining the head’s mechanical response to impact and ascertained the mechanisms associated with brain injury. As momentum and energy are transferred to the head during a direct impact, causing change in head motion, this leads to deformation of soft tissues involving the scalp, skull, and brain. Translational or linear head motion predominantly associates with focal injuries that are localized, and more severe in nature, such as intracranial bleeds and skill fracture. Focal injuries typically result from high-magnitude, short-duration loading, caused from pressure gradients throughout the brain and/or skull deformation. , As linear head acceleration/deceleration was originally found to highly relate to internal pressure changes, it became a primary metric in TBI research. This led to the development of integrations using peak linear acceleration and its time curve, specifically the Gadd Severity Index and Head Impact Criterion to evaluate safety performance of head protection. , These metrics are simple measures representing a single-axis resultant acceleration curve from impacts of one direction and location. The success of linear acceleration-based metrics is demonstrated in the ability of helmets to mitigate risk and reduce life-threatening injuries in contact/collision sport. Many challenges faced today in sport pertain to injuries caused by brain motion within the skull and the integrity of axons including SRC and cumulative RHI, which more closely associate with rotational head motion. , Holbourn initially suggested that shear strains within the brain are the main cause of injury. As linear acceleration forces produced compressional strains, they do not have an injurious effect because of the near incompressibility of neural tissue. They concluded that rotation had a far greater influence on brain damage than translation, and only shear stresses/strains were responsible for injury. Research later demonstrated this theory, showing that controlled rotational, or angular acceleration, was the primary source of shear strain and brain displacement capable of producing a range of injury severities including cerebral hematoma and diffuse axonal injury. , To address risks associated with SRC, a number of rotation-based injury risk metrics exist, which include peak rotational acceleration, peak rotational velocity, the Generalized Head Acceleration Model for Brain Injury Threshold (GAMBIT) and Head Impact Power (HIP). , GAMBIT was developed to consider both linear and rotational acceleration, and direct and indirect impacts in head injury criterion. HIP integrates directional components, which may be an advantageous target for safety standards helmet design ; however, because the final criterion established is a single value, it provides little explanation for the cause of any change (ie, impact location) and lacks predictive capacity, as there is no direct link to injury mechanism. Metrics that incorporate rotational head motion account for more variables; however, no one metric exists that sufficiently captures all relevant characteristics, including component head motion and time curve duration and shape. Due to the viscoelastic mechanical properties of neural tissue, damage may incur at low peak acceleration magnitudes with extended durations of dynamic loading. The brain is less resistant to rotationally induced shearing as the duration of the event increases. For example, a helmet may reduce the peak acceleration to a level deemed “low” risk; however, as energy attenuation is prolonged, the increased duration of loading leads to injurious tissue stress and strains. Although there have been many iterations of kinematics-driven brain injury predictor metrics, as a whole, they are not widely used in injury research. Their predictive capacity is limited and do not reflect the tissue level mechanisms of injury. With the development of improved computational power, finite element modeling of the human brain affords a detailed analysis of how kinematics affects brain tissue. The newest approaches incorporate tissue strain in their predictive metrics, such as DAMAGE (Diffuse Axonal Multi-Axis General Evaluation). Although these approaches are certainly improvements on past metrics, establishing a link between different levels of brain tissue and injury outcomes (ie, metabolic, structural) is complex.
Brain Tissue Deformation
Tissue deformation caused by head motion from direct impact is considered a primary measure in biomechanical head injury research. Sophisticated computational modeling provides an important and useful tool for measuring brain tissue response to ascertain relationships between local mechanical deformation and brain injury. Maximum principal strain (MPS) is a frequent brain deformation metric used in the biomechanical evaluation of injury prediction and risk, as it is in close agreement with anatomic failure testing and measurement of brain function. , MPS describes tissue elongation relative to its original length along one of the principal axes. A summary of strain values associated with a spectrum of head impact severities, injury risk, and outcomes are presented in Fig. 1 . A general severity scale can be observed from this research reflective of the range of trauma experienced in sport environments. Risk probability estimates to injury outcomes are calculated using logistic regression curves from combined injury and “no” injury data sets. A 50% risk probability of sustaining a concussion is estimated below 0.27 MPS within cerebrum gray and white matter, , , and trend toward lower estimates than reported average strain values. , High reported strains associate with risk of loss of consciousness and persistent post-concussion symptoms. , Lower strain values are documented from event reconstructions of asymptomatic impacts with no acutely identifiable/reported injury. , Kendo sword strikes to multiple head locations using a Hybrid III headform elicited a 0.05 to 0.07 MPS response, where no known reports of cumulative brain injury or abnormal rates of neurologic disorders are documented among practitioners. Clark and colleagues reported similar, yet a wider range, of strain than Kendo strikes from various forms of a lacrosse stick slash to both a protected (0.05–0.11 MPS), and unprotected head (0.06–0.15 MPS), a common cause of head injury in lacrosse, especially in woman’s leagues that do not require head protection. Research on lower-magnitude impacts is immature and therefore distinguishing and interpreting implications for risks on the playing field is preliminary. Low magnitude may be influential in cumulative event scenarios potentially leading to changes in neuronal physiology and/or structure. Karton and colleagues reported from a 32-game analysis that more than 90% of the head impacts experienced by lineman in professional American football (AF) caused strains ranging from 0.08 to 0.17. This level may correspond to changes in white matter microstructure and cumulative axonal injury measured in athletes following a season of play with no reported concussion. , Increasing evidence suggests that all magnitude levels within contact/collision sport should be considered, and therefore the full severity scale should be represented in overall exposure measurements.

Defining tissue response and neurologic injury
Axonal Strain
The increasing rates of mild head injury in collision/contact sport has initiated ongoing research that uses tissue cultures to link various levels of head motion to localized axonal stretch that disrupt the structure and function of cell membranes. An understanding of pathophysiological mechanisms associated with head injury outcomes is important in biomechanics research, as it provides a platform for establishing the human tissue’s biological response and tolerance criteria. Various axonal responses are observed from an applied strain, including swelling, electrophysiological impairment, morphologic responses, cytoskeleton disassociation, and axotomy. Studies demonstrate the brain’s biological response to mechanical loading, using strain as a measure of injury. A summary of strain values associated with functional and structural changes to the axon are presented in Fig. 2 . Responses may be acute and transient; however, may also initiate chronic cellular dysfunction and death. Axonal failure occurs in 2 ways: the primary mode is mechanical rupture and the secondary mode is gradual failure via progressive degradation, in which mechanical tissue strain has been linked to both in the in vitro TBI models. , Smith and colleagues demonstrated primary axotomy at 0.65 dynamic stretch, but when an axon is stretched below magnitude causing primary axotomy, morphologic changes develop, wavelike appearances caused by immediate breakage and buckling of microtubules, at periodic points along their length. Although axons will gradually recover their original prestretch straight orientation, these swellings are similar to those found in brain-injured humans. Physical damage from direct mechanical failure triggers progressive disassembly of microtubules around breakage sites. Low strains of 0.05 to 0.15 have been associated with functional impairment of signal transmission in the absence of structural damage. , , Yuen and colleagues used cell cultures to demonstrate 0.05 strain was the minimum injury threshold required to observe minor undulations and induce calcium influx. Further, significant increases in calcium were observed when 2 sub-threshold injuries were repeated within 24 hours. Weber demonstrated that the extent of cumulative cell damage in vitro was also dependent on the time between repeated injuries. Injured cultures experienced more neuronal loss and released more neuron-specific enolase and S100-B from repeated stretch induced within shorter time intervals. Ahmadzadeh and colleagues modeled macroscopic strains as low 0.05 causing microscopic changes in the form of protein unfolding, disrupting tau-tau bonding and tau-microtubule connections. Under dynamic loading conditions of rapid axon stretch, tau proteins behave more stiffly, resulting in primary breaking of microtubules, which has been shown to lead to subsequent disassembly of microtubules and axon degeneration.

Neuro-metabolism and Inflammation
Temporary neuronal dysfunction may express as ionic shifts with altered metabolism and inflammation. Both responses demonstrate a window in which the brain is vulnerable to second impact. A state of decreased cerebral metabolic rate of glucose (CMRglc) that occurs following an injurious impact worsens if a second insult is experienced before recovery. After metabolic recovery, however, CMRglc depression resembles that of a single injury. The precise duration of heightened vulnerability conceivably is not universally defined; however, 2 stretch injuries within 24 hours may exacerbate tissue injury. Subsequent insults during this time are often more severe, take longer to recover from, and the brain is less tolerable to impacts of lower severity. An interinjury interval of 120 hours and 144 hours has demonstrated sufficient time to avoid repeat injury. , Posttraumatic metabolic changes in the immature brain appear to be shorter lasting than in adults, but axonal vulnerability to injury may be more prominent in the young brain. Chronic consequences of RHI could lead to excitotoxic processes from repeated indiscriminate presynaptic release of glutamate. Neurons can be particularly vulnerable to glutamate receptor activation, , and higher levels of glutamate have been measured in athletes with a history of RHI. Further, a state of oxidative stress may lead to irreversible damage to neuronal membranes, causing secondary pathophysiological mechanisms leading to chronic neurologic deficits and cell death.
Similarly, when a second injury is experienced during the acute inflammatory phase, the repair process is halted and neuroinflammation is enhanced. However, if the secondary injury is experienced during the wound-healing phase, then normal processes continue. Persistent proinflammation, manifested by extensive activation of microglia and astroglia, associates with the onset of depression, and may contribute to posttraumatic neurodegeneration. Prolonged microglial activation is measured in active and retired athletes with a history of RHI and worsens brain disease pathology. Neuroinflammatory response to physical trauma may contribute to phosphorylated tau (p-tau) pathology, specifically in the dorsolateral prefrontal cortex in those diagnosed with CTE, where the degree of neuroinflammation associates with longer duration of RHI exposure.
Disruption of the Blood Brain Barrier
Blood brain barrier (BBB) dysfunction is observed in patients who have sustained severe TBI and milder forms of brain injury and RHI. , A breakdown of BBB components influences time course and extent of neuronal recovery, which may persist in individuals with RHI exposure. , BBB disruption allows peripheral immune cells to infiltrate into the brain, which leads to further complications of long-lasting inflammation and allows for the extravasation of plasma proteins into the brain. Research on RHI shows that extravasation of systemic components is specifically found in regions associated with distinct areas of perivascular p-tau deposition in patients with CTE, , a similar disease course in other tauopathies. Blair and colleagues demonstrated using an animal model that perivascular p-tau deposition caused BBB dysfunction, but with a suppressed tau buildup, BBB integrity was recovered. In clinical studies, elevated serum S100 B concentrations, a biomarker of BBB dysfunction, are detected in athletes exposed to RHI, including boxers, and soccer and football players. , Marchi and colleagues studied a cohort of 67 college football athletes reporting serum S100-B levels were highest in athletes receiving the highest number of head impacts, and S100-B antibodies predicted lasting changes in mean white matter diffusivity. BBB disruption has been reported as early as 2 hours after RHI in adolescent rugby players and associates with their level of exposure.
Neuroimaging
Subtle structural and/or functional abnormalities following head trauma can be identified using neuroimaging measurements. Changes within the microenvironment and axonal structure may contribute to long-term progressive changes and functional disturbances. Methodologies involving diffusion tensor imaging (DTI) show sensitivity in detecting microstructural damage via axonal mean diffusivity (MD) and fractional anisotropy (FA) in white matter integrity and gray-white matter junction after diagnosed mild brain injury, and asymptomatic RHI, , often involving the corpus callosum. , In several studies, changes in white matter structure and neuronal activity have been reported following a single season of play among athletes involved in contact/collision sport without diagnosed injury; in these studies, the frequency of head impacts is positively correlated with the extent of the measured changes. , , , Research regarding long-term RHI on white matter damage is immature; however, repeated mild head injury causes white matter thinning and reductions in myelin in animal model corpus callosum. Further, experiencing trauma during periods of neurodevelopment may affect multiple processes including myelination, demonstrated in altered corpus callosum microstructure later in life. Although less common to DTI, changes in brain activation following head trauma is measured using functional MRI (fMRI) in the form of cognitive task execution often involving working memory using auditory, visual, verbal, and motor performance. Neurophysiological fluctuations from baseline and post season whole brain and region of interest activation have been observed in athletes sustaining RHI. Higher-impact frequencies correspond with neurophysiological changes within various regions of the brain and associate with neurocognitive and neuropsychological outcomes in nonconcussed athletes. , For instance, the frequency of soccer headers associates with poorer test performance on psychomotor speed, attention and concentration, working memory, and memory. Hypoconnectivity during resting state and hyperactivation of brain regions during cognitive tasks has also been measured in retired athletes with pronounced deficits.
Cytoskeletal Disconnection and Biochemical Markers of Brain Injury
Axonal stretch may compromise the structural integrity of the cell through microtubule and neurofilament disruption. Various biomarkers have been associated with injury in patients with severe and mild TBI, and in symptomatic and asymptomatic athletes. , , Kondo and colleagues showed similar durations of elevated levels of pathogenic cis p-tau protein from repetitive trauma compared with single severe TBI, suggesting similar neurologic risks associated with repeated trauma to neural tissues. Serum biomarkers of axonal injury following single insult in ice hockey tend to return to baseline sooner (7–8 days) compared with repeated impact with knockout in boxing, which may take up to 36 weeks to return to baseline. Biochemical biomarker concentrations are higher in those experiencing higher RHI frequencies in multiple sports, predominantly, neurofilament light polypeptide and total-tau. , Di Battista and colleagues reported higher plasma-tau levels in male collision sport athletes (AF, ice hockey, lacrosse, rugby) to noncollision sport comparisons. Increased levels were also found in noncollision sport athletes, specifically following heading in soccer, which persisted for 1 month, and significantly correlate with number of headers. Higher plasma total-tau and exosomal tau levels are reported in retired AF athletes compared with controls. , In cases of CTE, neurofibrillary tangles are found throughout the brain tissue, indicative of continued disruption of microtubules and neurofilaments.
Fluid Exchange and Prion Propagation in Tauopathy
There is exchange between cerebrospinal fluid (CSF) and interstitial fluid (ISF) facilitating metabolite and waste clearance through fluid drainage pathways. Physical trauma affects this system, potentially leading to secondary pathophysiological mechanisms. Iliff and colleagues demonstrated that lymphatic pathway function is reduced following head trauma, persisting for at least 1 month after injury, and renders the brain vulnerable to tau aggregation. In CTE, p-tau deposition is initially found around perivascular spaces and along low-resistance interstitial pathways associated with CSF/ISF exchange. Even with cessation from participation, studies indicate that biomarkers within CSF may take months to return to normal. Elevated levels for weeks after trauma may lead to chronic levels of circulating waste, caused by continued RHI exposure without compensatory time for recovery. This potentially overwhelms flow and clearance leading to chronic impairment of glymphatic system, resulting in increased levels of interstitial tau. Further, impediment of the CSF and ISF flow and drainage might play a role in the prionlike spread of abnormal p-tau. Prusiner’s work with biogenic mice identified a disease progression from a self-propagating process of tau prions, suggesting that brain trauma may cause prionlike spread of pathogenic protein. Misfolded tau proteins cause structural disruption of nearby native tau proteins eventually inhibiting cell-to-cell communication. The movement of self-propagating proteins is a slow process, explaining why even the most aggressive cases of disease often express decades after trauma. ,
Quantifying repetitive head impacts using biomechanical metrics
Duration of Exposure and Cognitive Impairment
Duration of athletic career and years of RHI exposure significantly contribute to behavioral and cognitive abnormalities. , , Initially described in boxers, persistent poor mental health outcomes associate with the number of bouts and knockouts experienced. Self-reported fight exposure scores of years of fighting and fights per year associates with lower brain volumes and greater cognitive impairments in professional boxers and fighters. Similarly, cortical thinning in retired soccer players associates with decreased memory performance and RHI exposure. Jordan described risk for brain disease development and progression as high exposure, defined by career duration and age of retirement. In a convenience sample of deceased football players’ brains, McKee and colleagues found a positive correlation between years of contact sport participation and progressive stage of CTE. Interestingly, the same relationship was not found between the number of diagnosed concussions and pathologic progression. Vulnerability of young developing brains to cumulative RHI is demonstrated through positive associations in predicting neuropsychological and cognitive impairments later in life. Participation in collision sport increases one’s risk of depression, apathy, executive dysfunction, cognitive deficits, and lower mental functioning in adulthood, and participation at younger ages associates with greater deviations from normal functioning. , Similarly shown in noncollision sports, adult soccer players with the highest lifetime estimates of heading showed lower scores in attention and concentration, cognitive flexibility, and general intellectual functioning. Many current studies reporting the relationship between RHI exposure and cognitive impairment are limited by subjective measures of exposure from participant recall in relation to objective outcomes.
Cumulative Exposure Measurement Metrics
Few studies have developed a metric for cumulative RHI drawing from impact sensors data. A cumulative head impact index (CHII) is derived from impact frequency in conjunction with self-reported history measures of number of seasons played, position played, and level of play to estimate exposure in former professional AF players. CHII positively correlates with plasma-tau levels and the degree of neurocognitive deficits in former athletes. , Risk weighted cumulative exposure (RWE), a metric developed using concussion injury risk curves of peak head acceleration was used to measure impact exposure in high school football. Statistically significant linear relationships were found with RWE score and decreased FA after one season of play. Munce and colleagues measured the cumulative head impact exposure (HIE) defined as impact frequency (#) × impact magnitude of linear acceleration in youth football. Individual clinical measures of balance, oculomotor performance, reaction time, and self-reported symptoms of neurologic function were not correlated with HIE. Current indices estimating RHI provide limited information regarding event details (ie, mass, velocity, location, compliance), accurate head rotation, event duration, and strain, which are important for defining tissue response to trauma. ,
Impact Sensor Technology
Wearable technologies have become popular for measuring head dynamic response by placing sensors in helmets, mouth/chin guards, on skin, or worn as headband/cap. Sensors objectively measure exposure as impact frequency and acceleration magnitude during a game, practice, and/or season ranging from youth to collegiate levels within a number of sports, collecting large amounts of data in real-time. Methods using these devices have supported biomechanical characteristics such as impact frequency and density as influential variables to neurobiological and neurocognitive outcomes. Many studies have drawn from various modalities in conjunction with sensors to identify associations between a measured change within the brain and impact frequencies outside the boundaries of acute clinical expression. , , , Bazarian and colleagues demonstrated that changes in white matter microstructure, specifically greater voxel percentage with decreased FA, was significantly correlated with head hit frequency throughout a season exceeding 2 magnitude values of peak rotational accelerations; impacts greater than 4500 rad/s2 exceeded 30 to 40, and impacts greater than 6000 rad/s2 exceeded 10 to 15. Information collected using these technologies is used by sports organization, coaches, and parents to support decisions about managing accumulated brain trauma. As an evaluation tool, sensors are limited in providing quantifiable evidence for the effectiveness of an intervention designed to decrease exposure. Head accelerations measured from helmet sensors during illegal collisions (23 g; 1530 rad/s2) were reported as significantly higher when compared with those considered legal (21 g; 1417 rad/s2); however, these differences provide limited information concerning safety, risk, and clinical relevance. Evaluation of a helmetless-tackling football practice training intervention demonstrated an average of 30% fewer impact frequencies per athletic exposure compared with controls. Their analysis showed this reduction happened at 2 time points, week 4 and week 7, and disappeared by the end of the season. This analysis provides quantifiable information on decreased frequency; however, the decrease may have been influenced by multiple factors not limited to helmetless-tackling practice. Valid concerns have been raised regarding the accuracy of sensor technology for measuring dynamic magnitudes and duration, particularly evident for rotational head motion. Accurate measurements of rotational motion, a variable closely associated with brain tissue strain, is important when measuring brain trauma. Issues with helmet fit, sensor/skull coupling, and oblique impact events add further complication. , There is value in their relative readings, where higher magnitudes are interpreted as sustaining higher trauma, but connecting biomechanical measurements to the injury is difficult, as current technologies lack accuracy in predicting brain tissue response, consequently making exposure results difficult to interpret and challenges their usefulness as a stand-alone measurement tool for exposure.
Primary Characteristics that Associate with Neurologic Injury
Various forms of neurobiological and neurocognitive changes consistently demonstrate recurrent associations with tissue strain magnitude, the frequency of damage, the time interval between events, and duration of exposure. Trauma-induced cellular changes are observed in athletes following symptomatic and asymptomatic head trauma, , , , and the degree of alteration associates with the impact intensity and frequency. , , Moreover, time for neurobiological recovery from either frequent or intense impacts has been implicated in recurrent injury and brain health. , , , Exposure to RHI is not uniform and is created using various combinations of these characteristics. Brain trauma profiling (BTP) is an objective approach to quantifying exposure by measuring and incorporating these primary characteristics that associate with neurologic risks. Strain magnitude, impact frequency, time interval between impacts, over a specified duration defines cumulative RHI exposure, and has effectively described subtleties between exposure profiles within multiple sports and ability levels. A metric, brain strain exposure over time (BSE/T), calculated using these characteristics distinguished player field position in professional AF, which identified 3 statistically unique profiles, a reflection of their different environmental conditions ( Fig. 3 ). Tight end was identified as a hybrid position of both lineman and short distance receiver, illustrating the sensitivity of BSE/T. AF players are at high risk for the spectrum of acute and chronic neurologic injury, , , making them an important subset of individuals for establishing dose-response relationships between exposure in an uncontrolled environment (game play) and risk to various outcomes. BTP can be a useful tool in facilitating this knowledge. This approach describes differences in exposure profiles for individuals, teams, sports, age levels, gender, and competitive level, providing a platform for making informed decisions on effective risk-reduction practices. Table 1 presents the frequency and magnitude of head-to-ice and head-to-shoulder impacts documented in youth ice hockey. This analysis provides detailed objective evaluations of head contact characteristics that is valuable in establishing the most impactful intervention efforts for risk-reduction outcomes specific to level of play. Safer play can be achieved by effectively managing 1 or more trauma characteristic. How these characteristics are managed need to be based on the exposure profile, contextual definitions leading to contact, and appropriate modification strategies for the sport application.
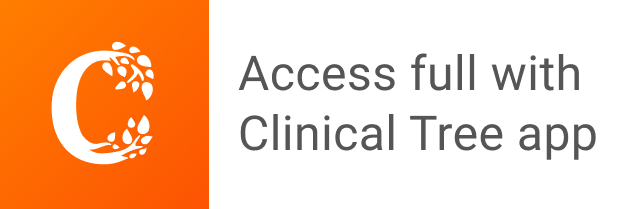