Key points
- •
Intervertebral disc (IVD) degeneration involves endplate-driven and annulus-driven phenotypes that accumulate and interact with progressive degeneration and eventual collapse of the IVD.
- •
IVD injuries result in organ-level changes that affect cellular level responses and organ level biomechanical behaviors, which can be measured and manipulated in model systems.
- •
IVD degeneration phenotypes can be represented by distinct injuries in biomechanical model systems including endplate-driven defects and annulus-driven defects, as well as biochemical degradation.
- •
Annulus-driven and endplate-driven IVD injuries, and biochemical degradation, can all result in depressurization that biomechanically results in IVD height loss, and affect low force behaviors such as an increase in the neutral zone.
- •
Large annulus-driven defects create a loss of annulus fibrosus integrity and affect torsional stiffness and can also increase torsional range of motion in addition to resulting in depressurization.
Acknowledgments
We gratefully acknowledge Christopher Smith, MA, CMI, Academic Medical Illustrator, Icahn School of Medicine at Mount Sinai, for his medical illustrations that greatly contribute to this chapter, and Byron Mui, BA, for helpful discussions on biomechanical model systems.
Funding
This work supported by NIH/NIAMS Grants 1 R01 AR069315,1R01 AR064157, and 1R01 AR057397.
Introduction
Back and neck pain involve pathologies of all spinal tissues that result in functional changes to spinal biomechanics and biology. As spine research advances, it has become clear that many clinical definitions of spinal pathologies have limited relationships to painful conditions, motivating a need for more precise phenotypic characterizations. One of the most clear examples of this is intervertebral disc (IVD) degeneration (see Chapter 6 ), since abnormalities in disc magnetic resonance imaging (see Chapter 5 ) are common in asymptomatic controls and do not always correlate with clinical symptoms in patients [ , ]. Consequently, IVD degeneration is commonly considered to have similar characteristics of aging and often considered an aging phenomenon and is accelerated in some [ ]. More precise definitions of functional pathological phenotypes are required to describe better the pathological changes associated with disability and pain and to distinguish those changes from aging phenomena. Modeling these specific phenotypes for biomechanical testing is therefore critical to the study of spinal pathology
The purpose of this chapter is to describe biomechanical models to study spinal phenotypes, which include tissue and structural biomechanical models, cell and organ culture, and finite element modeling. We first describe IVD degeneration phenotypes and then describe the biomechanical, and to a lesser extent the biological, changes involved in distinct injuries that simulate these spinal pathology conditions. We describe findings that have informed pathophysiology known to be associated with IVD degeneration to clarify important biomechanical characteristics required to repair these degenerative conditions.
Intervertebral disc degeneration phenotypes
The IVD consists of a central nucleus pulposus (NP) surrounded on its periphery by the annulus fibrosus (AF) and on the superior and inferior surfaces by the cartilaginous endplates (see Chapter 1 ). The IVD is part of a spinal organ system, where the IVD and vertebral tissues interface closely at the cartilage and vertebral endplates. The outer AF is in contact with ligamentous and tendinous tissues that further support the mechanical function of the spine allowing its stability and motion.
Intervertebral disc degeneration has been described as the process of chronic, progressive structural failure with frustrated healing and frequent reinjury [ , ]. Injury and healing are both mechanically modulated so that immobilization and its contributions to remodeling are as important as overloading in contributing to this vicious cycle of frustrated healing [ ]. The poor nutritional environment and increased glycation of IVD matrix proteins amplify this poor healing response in IVDs, and increased catabolism and protein aging increase the susceptibility of IVD tissues to structural failure in aged IVDs.
A single term of IVD degeneration is not satisfactory to define the multitude of complex processes and interacting conditions commonly associated with IVD degeneration. Many distinct initiators of IVD injury can lead to progressive degeneration with a common end result of IVD structural failure and collapse commonly referred to as IVD degeneration. Refined phenotypic descriptions, therefore, are more important in defining earlier degenerative states, where improved understanding and timing of IVD related diseases may point to clearer diagnostic criteria, definitions, and earlier interventions for painful and disabling spinal conditions.
Two distinct clinical IVD degeneration phenotypes have been proposed to describe IVD structural failure: endplate-driven and annulus-driven IVD degeneration [ ]. The endplate-driven phenotype is characterized by endplate defects and inward collapse of the AF. This phenotype is associated with high heritability and mostly affects IVDs in the upper lumbar and thoracic spine. These conditions often are associated with compressive injuries, such as a fall on the buttocks, which start before the age of 30 years, and lead to moderate back pain. Annulus-driven IVD degeneration typically involves a radial fissure and IVD prolapse, has a low heritability, and mostly affects IVDs in the lower lumbar spine. This condition is associated with repetitive bending and lifting, commonly develops progressively after age 30 years, and leads to severe back pain and sciatica.
Cytokines and the inflammatory system play strong roles in the healing of all tissues, yet exposure to high amounts of proinflammatory cytokines to immunoprivileged IVDs can result in painful degeneration, which itself is also considered to include a chronic inflammatory state and extensive matrix catabolism [ , ]. As a result, biochemical degradation and accompanying inflammation are other important components of acute IVD injury and are also commonly seen in chronic IVD degeneration.
Just as changes in IVD degeneration can be characterized into distinct phenotypes of structural failure, the injury models intended to simulate IVD degeneration processes can be organized into distinct injury types that result in specific functional biomechanical and biological changes. Such IVD injury types are often distinct in early stages of degeneration, interact in moderate stages, and eventually involve substantial damage accumulation and tissue deterioration as they evolve into advanced stages of degeneration with IVD collapse. Describing the specific IVD injury models allows for a more precise understanding of the changes they impart on the IVD and informs how they may interact with the other injuries and damage involved in the more complex clinical phenotypes. The following IVD injury types directly relate to commonly used models that simulate IVD injury and degeneration ( Fig. 3.1 ):
- 1.
Endplate-driven defects
- 2.
Annulus-driven defects

Both of these defects can induce biochemical changes that degrade and destroy the NP and its extracellular matrix resulting in depressurization, which is a functional change causing biomechanical instability that is common to multiple IVD degeneration phenotypes. Furthermore, biochemical degradation affecting the NP can predispose other IVD tissues to injury. As a result, biochemical degradation must also be considered when describing IVD injury types because they are an important driver of depressurization and a typical feature in multiple IVD degeneration phenotypes. Understanding each of these IVD injury types and biochemical degeneration requires an interdisciplinary approach to complex and interacting factors and tissues.
Intervertebral injury models
Injury model systems are simplifications of degeneration processes that offer greater control of specific interventions. As a result, both normal and injured IVDs must be tested, and the injuries must be induced in a consistent manner to reduce variation and better elucidate the role of injury, as compared to the more complicated processes of IVD degeneration. Endplate-driven injuries commonly include endplate fracture or other endplate changes that can inhibit the transport of nutrients and bioactive substances into and out of the IVD. Annulus-driven injuries include needle puncture, lacerations to the AF, and nucleotomy procedures. Model systems for each include biomechanical testing systems on IVD motion segments or tissues, cell and organ culture models, and computational models such as finite element modeling (FEM). However, before discussing specific injury models, we must have a basic understanding of biomechanical testing strategies.
Introduction to biomechanical testing strategies
Biomechanical models are used to study the IVD response to loading and can be used to identify and characterize IVD degeneration phenotypes (see Chapter 2 ). This is often done on explanted motion segments from either animal models or human cadavers. IVDs of large animals commonly have greater biological and biomechanical similarities to humans than IVDs from small animal models, yet several studies have described ways to compare IVDs across different species with human IVDs by comparing effective stresses or by normalizing properties by other geometric parameters [ ]. Spinal biomechanical studies on single motion segments provide knowledge of how distinct injuries may impact a single vertebra-IVD-vertebra unit while biomechanical tests on longer spine specimens with multiple motion segments can better assess the role of ligamentous structures surrounding the spine and better simulate the spinal organ [ ]. In this case, muscle loading must be simulated to maintain stability, and lumbar spine segments can support compressive loads of physiologic magnitudes without collapsing if the load is applied along a path that follows the centers of rotation of the lumbar segments, or a follower load path [ , ].
To perform mechanical testing, motion segments are fixed at each end and placed in materials testing machines which can impart a variety of loads onto the segment. Methods of fixation vary and may include potting into dental cement, grips, or clamping devices [ ]. Specimens should be kept moist during testing with physiologic fluids, such as phosphate-buffered saline to avoid desiccation and resulting, unintended mechanical changes [ ].
Loads may be imparted in a variety of deformation modes, including compression, tension, flexion, extension, bending, shear, and torsion ( Fig. 3.2 ). Pure moment tests are commonly applied to assess flexibility and neutral zone of motion segments [ , , ], as are displacement-controlled stiffness tests [ ]. These can be applied individually or in combinations.

Mechanical testing is used to measure the nonlinear biomechanics of the IVD. A typical nonlinear curve has three distinct regions: two linear regions of gradually increasing slope at the tip and tail of the curve, and one interior region of low slope, known as the neutral zone. The characteristic mechanics of the disc are summarized with the following parameters: total range of response, neutral zone length, and stiffness of the three regions. The range of response is the total motion or load developed in the disc and can have units of displacement, rotation, force, or torque. The neutral zone is the range of motion with minimal resistance to motion [ ]. It is represented by the flat part of the curve and has units of displacement or rotation ( Fig. 3.3 ). Stiffness is the measure of resistance to displacement or rotation and has units of load/displacement or torque/rotation.

Intervertebral disc height loss is the total change in IVD height and has units of displacement or, when normalized to original height, percentage. Pressure is the measure of the hydrostatic load inside the NP. Hysteresis is a measure of energy absorbed by the IVD and is used to describe viscoelastic behavior. Strain is used to describe deformation with respect to the original dimensions of the IVD and is dimensionless.
Endplate-driven defects
The cartilaginous and bony vertebral endplates serve mechanically to distribute pressure evenly across the IVD and to promote and constrain flow into/out of the IVD [ ]. Cartilage and vertebral endplate structures abut and interact, and both undergo large changes with aging and degeneration. Schmorl’s nodes, Modic change, and endplate thickening are some of the clinically observable endplate changes that are considered to affect stress distributions and transport in motion segments (see Chapters 10 and 11 ) [ ].
Endplate disruption and dehydration in human cadaver bending models have led to a reduction in IVD height as well as an increased neutral zone ratio [ ]. Further, it has been demonstrated that endplate fracture can initiate IVD degeneration by creating abnormal stresses on the adjacent IVD [ ]. Intervertebral disc organ culture has been used to model traumatic endplate damage by inducing a burst fracture, and these experiments demonstrate a consistent decompression and herniation of the NP through the endplate as well as several biologic changes, including both AF and NP cell death [ ]. In a follow-up experiment, a comparison of the impact load causing burst fracture to an equi-energetic load demonstrated that the presence of the burst fracture itself leads to degeneration of the IVD [ ]. These injury models demonstrate how endplate defects have the potential to initiate and drive degeneration of the IVD. The concept that structural defects result in apoptosis and loss of cellularity has also been shown for delamination injuries in the AF [ ].
Another approach, FEM, has been utilized as a method of understanding the biomechanics of the IVD in both normal, degenerate, or injured states. Finite element modeling utilizes the idea that complex systems can be broken down into many small simple components, or elements, that are more easily solvable and can be computationally combined to better understand and simulate problems of complex physics and geometry. In fact, FEM is a common tool in biomechanical modeling of the spine used to investigate several variables that may include the geometry of the IVD, changes in water and proteoglycan content, mechanical properties of the AF and NP, and more. Natarajan et al. [ ] developed a FEM of a motion segment without posterior elements to study the initiation and progression to failure in motion segments and found that failure started in the endplate and not in the annulus, highlighting the role of the endplate in IVD injury and degeneration. This work is consistent with the finding that endplate junction failures are common in clinical herniations [ ].
The endplate plays a unique dual role by not only distributing compressive loads across the IVD but also acting as a mediator of solute transport [ , ]. Experimentally, lack of glucose and accumulation of lactic acid in nutritionally compromised IVDs is a cause of IVD cell death [ , ]. Compounding these problems, degeneration can lead to an increase in endplate cross-sectional area [ ] and decreasing numbers and sizes of small holes in the endplate that correspond to capillary bud contact channels [ ]. Dynamic loading can increase IVD cell metabolic demands and interact with nutrient compromise to increase IVD cell death under rigorous loading conditions [ ]. Finite element modeling simulation has also shown that dynamic loading can enhance the diffusive transport of nutrients to increase cell viability [ ].
Rather than a distinct injury event, endplate thickening can lead to nutritional compromise which can drive IVD degeneration by causing progressive cell loss and also by causing native IVD cells to become quiescent and produce less matrix [ , ]. It is possible that reduced transport across the endplate may not be a direct driver of degeneration, but rather a consequence of other biochemical and cellular signals that cause IVD cells to be less functional, perhaps producing ectopic calcifications [ ]. Tensile properties of the cartilage endplate are sensitive to changes in biochemical composition, and this relationship between tensile modulus and collagen/glycosaminoglycan (GAG) ratios changes near endplate defects [ ]. Consequently, degenerative changes are likely to make the endplate more susceptible to fracture, microfracture, nutrient compromise, or other susceptibility to IVD degeneration.
The morphological changes in the endplate thickness, porosity, and biomechanics are known to correlate with both aging and degeneration making their distinction difficult [ ]. However, more recent findings from the microstructural analysis suggest an alternate finding that endplate porosity increases with degeneration. Specifically, micro-CT analysis of degenerated cadaver IVD explants has suggested that with increasing age and degeneration, the endplate may become progressively more porous [ , ].
Overall, it is clear that nutrition and metabolite transport play crucial roles in the metabolic process of healthy and degenerative IVDs, and endplate changes can involve multiple competing processes and accelerate IVD degeneration [ ]. While reduced transport is likely to make IVD cells vulnerable to cell death and IVD degeneration, the reduced cellularity in IVDs with degeneration is also likely to occur partly from injurious loading and endplate defects. Nutritional changes can lead to cell death, though there are likely to be many other metabolic changes to IVD cells from these changes that can induce progressive changes to IVD structure, function, and failure properties. Understanding these distinct characteristics leads to the use of a narrow and accurate description of endplate defects as contributing to the endplate-driven phenotype.
Annulus-driven defects
Mechanically, the AF serves the roles of restraining NP swelling and connecting adjacent vertebrae [ ]. The AF collagen network is particularly good at resisting tensile stresses on the IVD due to the large collagen structures and the elegant connectivity with the vertebral body [ ]. Tensile strains in the AF arise due to hoop stresses (or the force exerted circumferentially in both directions on all particles in a wall of a cylinder) from NP swelling during axial loading and develop in larger magnitudes due to bending and shearing of the motion segment [ ]. The AF fiber orientation also allows it to play a strong role in resisting torsion [ , ].
Together, the literature suggests that torsional changes are sensitive and specific to AF integrity [ ]. For example, van Deursen et al. [ ] demonstrated that even small torsional rotations onto an in vitro porcine IVD model resulted in an increase in stress in the AF with a simultaneous decrease in stress in the NP. Michalek et al. [ ] found that annular injuries directly affect the torsional biomechanical properties of the motion segment in a manner proportional to the size of the injury. Human cadaveric motion segments were tested in torsion by Krismer et al. [ ] who demonstrated that increased torsional loading and IVD height loss were associated with fissure formation at the AF. Clinical literature shows some evidence for increased axial rotation in patients with IVD degeneration and concordant back pain, suggesting a loss of AF integrity and torsional instability [ , , ].
Needle puncture is one form of AF injury model, which involves inducing injury into an IVD ( Fig. 3.4 ) and can be divided into small and large injuries based on the gauge of the needle used to induce the injury. Needle puncture was first used in rabbit models as a means of producing a slow, repeatable IVD degeneration phenotype, and the degeneration severity increased with needle gauge [ , ]. Elliott et al. [ ] quantified the effect of needle gauge across species and identified that larger AF injuries where needle size was greater than 40% of IVD height led to degenerative changes across species, whereas smaller gauge needle puncture (≤40% of IVD height) injuries led to variable, more minor effects with a predominant reduction in NP pressurization noted. Along with the percentage of IVD height, the severity of the AF injury can also be quantified by the depth of puncture. Shallow injuries induce incomplete AF tears without NP expulsion and deep injuries through the AF wall thickness induce complete AF tears with NP herniation. Lai et al. [ ] found more significant injuries in deep versus shallow punctures as demonstrated by loss of IVD height. Injecting fluid into the IVD can induce more damage than puncture alone, including delamination with injection of saline [ ]. This has been shown clinically with discography in which Carragee et al., [ ] in a 10-year matched-cohort prospective study, demonstrated that discography is a “catalyst” for accelerated IVD degeneration, herniation, IVD height loss, signal loss, and the development of reactive endplate changes compared to noninjected IVDs.

Larger AF defects are simulated via a controlled surgical incision or punch that can vary in size, shape, and location, including rim lesions, midplane tears, radial tears, and concentric tears [ ]. These defects can be simulated via surgical injury to IVD cadaver explants or organ culture explants in vitro and via animal injury in vivo. Rim lesions are defined as a transverse defect near the attachment of the annulus to the bone of the vertebral body rim. Injury models of rim lesions have involved ovine lumbar IVD explants injured via surgical incision at the location of the annulus endplate junction [ , ]. Concentric or circumferential tears are described as a separation of the annulus lamellar layers, and injury models used here involve ovine functional spinal units injured via needle puncture and subsequent fluid injection to create a separation between the lamella of the annulus [ , ]. A radial tear is defined as being transverse to the lamellar fibers and injury models involve inducing injury via scalpel incision in explanted cadaveric IVD units [ , ]. These injuries can all induce depressurization as with smaller AF defects to include IVD height loss and low force biomechanical behavior changes. Large AF defects also produce a loss of AF integrity that can result in a loss of torsional stiffness or increased torsional range of motion [ ]. Taken as a whole, these studies demonstrate that acute, localized injuries have immediate, measurable effects on local IVD tissue and lead to measurable biomechanical changes.
Nucleotomy is another technique utilized to induce injury to an IVD. Frei et al. [ ] used human cadaveric specimens to measure deformation patterns in both compression and shear both before and after nucleotomy. This injury was found to result in decreased IVD pressure and endplate strains in compression, as well as decreased IVD pressure in shear, in keeping with the hypothesis that axial compression is closely connected to NP pressure. Showalter et al. [ ] showed that a partial nucleotomy performed via a cruciate incision decreased the compression and neutral zone modulus while increasing strain. Interestingly, nucleotomy had little effect on IVD mechanics after 10,000 cycles of cyclic loading, indicating that the NP was important in low force behaviors and for swelling and load redistribution required to restore mechanical behaviors following cyclic loading events [ ]. Overall, both small and large AF defects, as well as nucleotomy, serve as in vitro simulations of IVD injuries consistent with the annulus-driven phenotype of IVD degeneration.
Biochemical degradation: a common driver of IVD depressurization
Intervertebral disc depressurization is involved in annulus-driven and endplate-driven defects with or without loss of AF integrity. IVD cells produce large proteoglycan molecules that aggregate to form proteins with high densities of GAGs. These large structures maintain a high negative charge density and attract water into the NP, creating a large osmotic pressure which allows the NP to swell in response to compressive loads from the adjacent endplate. The high GAG content of the NP also is enmeshed within a loose matrix of randomly oriented collagen type II, which together creates an isotropic material that only loosely constrains these swelling pressures. These swelling pressures are therefore better constrained by the large hoop stresses generated in the AF. There can be a loss of NP pressurization for both endplate and annulus-driven degeneration, or by degradation of these GAGs. Endplate cracking increases the NP volume area, thereby reducing NP swelling pressure. AF defects also reduce NP pressurization with an increase in NP volume into the AF space or a loss of NP tissue via herniation and/or nucleotomy ( Fig. 3.5 ). Depressurization has been proposed to occur after small AF puncture injuries by creating a new fluid transport pathway, or “pressure vent” where injuries did not result in NP tissue loss nor NP volume increase [ ].
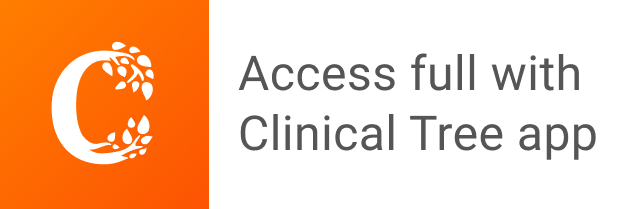