Chapter Preview
|
|
|
|
|
|
|
|
Surgical fusion of the cervical spine is a common procedure with a myriad of indications. Historically, allograft and autograft bone have been the mainstays of cervical fusion; however, in more recent years, surgeons have begun using a class of bone graft substitutes known as biologics. The biologics include demineralized bone matrix (DBM), ceramics, allografts containing mesenchymal stem cells, and growth factors. Each of these biologics has different characteristics and different degrees of osteoconductivity, osteoinductivity, and ostegenicity. This chapter reviews the key basic science principles, preclinical studies, and clinical studies that guide the surgeon in the appropriate use of biologics in the cervical spine.
Spinal Fusion
For successful spinal fusion to occur, sufficient bone graft, adequate vascularity to the fusion bed, and mechanical stability of the fusion levels are required. Without these components, the rate of nonunion is markedly increased. A key component of the fusion cascade begins at the cellular level. Progenitor cells, which respond to the local environment to produce bone, are the ultimate throughput allowing fusion to occur. These cells can be provided by cellular grafting to the fusion site, as well as through vascularity to the fusion bed.
A sufficient vascular supply to the fusion bed is required to complete the various phases of fusion biology. Adequate vascularity allows a hematoma, rich with various activated growth factors, to accumulate at the fusion bed. The factors ultimately attract progenitor cell migration into the fusion bed. These cells can then differentiate into osteoblasts.
The oxygen tension in the fusion bed also depends on blood supply. Investigators have hypothesized that an abundant blood supply leads to a higher oxygen tension level, which favors osteoblastic differentiation rather than chondrogenic differentiation. Following differentiation, the osteoblasts produce osteoid matrix, which calcifies into bone. The newly formed bone ultimately undergoes remodeling according to Wolff’s law, such that reorganization is based on the imposed load.
Mechanical stability is the final requirement for fusion. In bony fracture healing, Perren proposed the interfragmentary strain theory, which states that the amount of strain in the fracture gap determines the type of tissue formed during healing. Strain greater than 100% would lead to nonunion, 10% to 100% would lead to initial fibrous union, and 2% to 10% would lead to cartilage formation and endochondral ossification. Strains less than 2% would lead to direct bone healing.
Furthermore, once tissue forms, the fracture gap could stiffen, which would lower strain and possibly change the tissue characteristics in the gap. Similarly, in fusion healing, a rigid structure is needed to promote the formation of bony tissue rather than a fibrous union. Instrumentation in the spine helps promote bone formation by reducing the “fracture” gap strain between the functional spinal units. These findings suggest that a certain amount of rigidity in fixation may facilitate spinal fusion.
Historical Fusion Rates
Anterior Procedures
One of the most common cervical procedures performed is anterior cervical diskectomy and fusion (ACDF). ACDF has been highly successful in the treatment of radiculopathy, myelopathy, and myeloradiculopathy secondary to disk herniation and cervical spondylosis; however, the risk of nonunion following ACDF remains a real concern in certain patient populations. Fusion rates in the literature vary because no standard radiographic criteria exist for determining osseous fusion. Furthermore, successful fusion depends on the number of levels, graft type, and use of instrumentation. The fusion rates for single-level ACDF are much higher than for multilevel fusion. More specifically, for one-, two-, and three-level fusions, the fusion rates are 82% to 100%, 73% to 80%, and 70%, respectively. The use of allograft or autograft bone may also play a factor in the fusion rates, but in single-level fusions, this difference appears to be minimal. Instrumentation plays a critical role in fusion, particularly in multilevel constructs, to stabilize the motion segment and prevent graft compression and graft expulsion ( Fig. 48-1 ).

Posterior Procedures
Historically, posterior procedures have yielded high fusion rates. Rates of fusion using iliac crest autograft range from 20% to 100%, depending largely on the segments incorporated, the techniques used, and how fusion was assessed. In contrast to anterior surgery, location with regard to the cervical level being addressed plays a pivotal role in fusion rates in the posterior cervical spine. Occipitocervical constructs using iliac crest bone graft fuse 62% to 100% of the time, whereas atlantoaxial fusion has demonstrated pseudarthrosis rates with iliac crest–based constructs that exceed 40% in some series. Overall, a rate of 80% is quoted for successful atlantoaxial fusion. In contrast, for noninstrumented subaxial fusion procedures, fusion is reported in 92% to 100%, with a composite fusion rate of 97%.
As in anterior surgical procedures, the type of graft also plays a role in posterior procedures. Sawin and colleagues reported a fusion rate of 98.7% with autogeneic rib graft compared with 94.2% with iliac crest. The investigators speculated that the rib’s morphology contoured well to the cervical lordosis and may have allowed a higher fusion rate. In addition, investigators have hypothesized that a higher concentration of bone morphogenetic protein (BMP) may be found in the rib. Again, instrumentation plays a key role posteriorly because rigid internal fixation has been more successful than wiring constructs.
Summary
With these concepts in mind, a key difference in anterior and posterior cervical surgery exists when considering which type of bone graft is required. Whereas anterior cervical surgical procedures require the bone graft to resist axial compression, structural support is not a required function of bone graft typically used for posterior fusion. Axial compression support is usually needed in anterior surgical procedures because disk material is removed between the functional spine units, thereby destabilizing the spinal anterior column. The gap formed must be structurally supported to prevent the disk space from collapsing.
Furthermore, anterior cervical graft must have sufficient structural integrity to handle the axial loads without fragmenting, to prevent nonunion or malunion between the functional spinal units. This structural support is typically provided by the cortical component of the bone graft. If purely noncortical bone is used, then a structurally sound device (e.g., a cage) should be used. In contrast, with posterior cervical fusion, the structural integrity of the spine is typically intact (the anterior column remains untouched, and the lateral masses remain intact); therefore, axial loading of the graft is usually not a problem.
Definitions
Each graft material differs in its properties of providing a supply of osteogenic cells, an osteoconductive matrix, and an osteoinductive signal ( Table 48-1 ). The osteogenic property of bone graft refers to the cellular component of the graft that participates in bone formation. As such, this property typically refers to osteoblasts or osteoblast precursors such as stem cells or preosteoblasts.
Material | Osteogenic | Osteoinductive | Osteoconductive | Structural Support |
---|---|---|---|---|
Autogenous cancellous bone | + | − | + | − |
Autogenous cortical bone | + | − | ± | + |
Allograft cortical | − | − | ± | + |
Allograft cancellous | − | + | − | |
Demineralized bone matrix | − | + | + | − |
Ceramic | − | − | + | ± |
BMP | − | + | − | − |
Osteoconductive properties refer to the structural properties of the graft matrix that enhance the attachment, migration, proliferation, and differentiation of stem cells and other cells that contribute to bone healing. Porosity is an important aspect of osteoconductivity. Appropriate pore sizes ranging from 200 to 500 μm are thought to be ideal. The composition of the graft matrix can also affect the osteoconductivity. For instance, with biodegradable grafts, the degradation properties can alter osteoconductive properties.
Osteoinductivity refers to the ability of some substance, usually a growth factor, to stimulate cellular events that transform a potential cell into a differentiated cell that becomes activated and committed to contributing to new bone formation. Marshal Urist defined osteoinductivity by the ability of a substance to form bone at an ectopic location such as fat or muscle tissue. Known naturally occurring osteogenic factors include a subset of the transforming growth factor-β (TGF-β) gene superfamily and some BMPs. The most osteogenic BMP molecules are thought to be BMP-2, BMP-6, and BMP-9. Less osteogenic molecules can form bone at sufficiently high doses, and other BMP molecules do not form bone reliably even at very high doses. Many other growth factors that cannot form bone by themselves but may participate in bone formation TGF-β, insulin-like growth factors, and basic fibroblast growth factor.
In addition to classifying bone graft substances by their osteoconductivity, osteogenicity, and osteoinductivity, another very useful method of describing graft materials is based on their use as bone graft extenders, enhancers, or bone graft substitutes. Graft extenders are substances that, when added to autogenous bone, allow for fusion of more levels or the use of smaller amounts of autogenous bone to produce fusion equal to that of autogenous bone graft alone. Therefore, graft materials that are extenders must be used with a certain amount of autograft to be effective. In contrast, graft enhancers allow for a higher rate of fusion when they are added to autogenous bone as compared with autogenous bone graft alone. Therefore, graft enhancers must also be used with autograft. Bone graft substitutes completely replace autogenous bone and produce equivalent fusion rates. Therefore, by definition, substitutes do not require any autograft bone at all. These terms are valid for only the indication and situation in which the graft material was tested. For instance, rhBMP-2 can serve as a bone graft substitute for anterior lumbar interbody fusion in certain situations; however, the same concentration of rhBMP-2 in the same carrier sponge is ineffective as a bone graft substitute in posterolateral lumbar spinal fusion.
Traditional Bone Grafts
The most common traditional bone graft is an autograft, typically harvested from the iliac crest. These grafts combine osteogenic, osteoinductive, and osteoconductive properties. Autografts can be either cancellous or cortical. Cortical grafts provide mechanical stability, but with less osteoconductivity and fewer osteogenic cells than cancellous graft. Cancellous grafts provide an excellent osteoconductive matrix and more osteogenic cells that can enhance fusion biology, but they have poor structural integrity. Tricortical iliac crest bone, the most popular autograft, has a combination of the useful properties of cortical and cancellous bone and therefore is an excellent choice for anterior cervical fusion. However, autografts have certain disadvantages. These include a limited supply, donor site morbidity, increased operative time, pain, blood loss, increased risk of infection, risk of cutaneous nerve damage, and even a small risk of local fracture.
The use of allograft was popularized to avoid some of the potential pitfalls associated with the morbidity of autologous bone graft harvest. Furthermore, allograft can be pre-processed into various physical shapes and forms, thus optimizing the architectural properties and making it easier for the surgeon to use intraoperatively. Currently, cortical allograft is used for ACDF. In this situation, the allograft has neither osteoinductivity nor osteogenicity and has very poor osteoconductivity. Nevertheless, for a single-level ACDF, allograft bone has been shown to be highly effective, with fusion rates similar to those with autograft bone.
Some disadvantages of allograft include limited supply as a result of limited donor availability, religious and cultural inhibitions, and the theoretical risk of disease transmission. Furthermore, allograft bone is probably not as effective as autograft for multilevel fusion. The reason may be due the absence of osteogenicity of the allograft or possibly decreased host biologic acceptance compared with autograft. However, immunogenicity is typically not considered an important factor for cortical processed allograft bone.
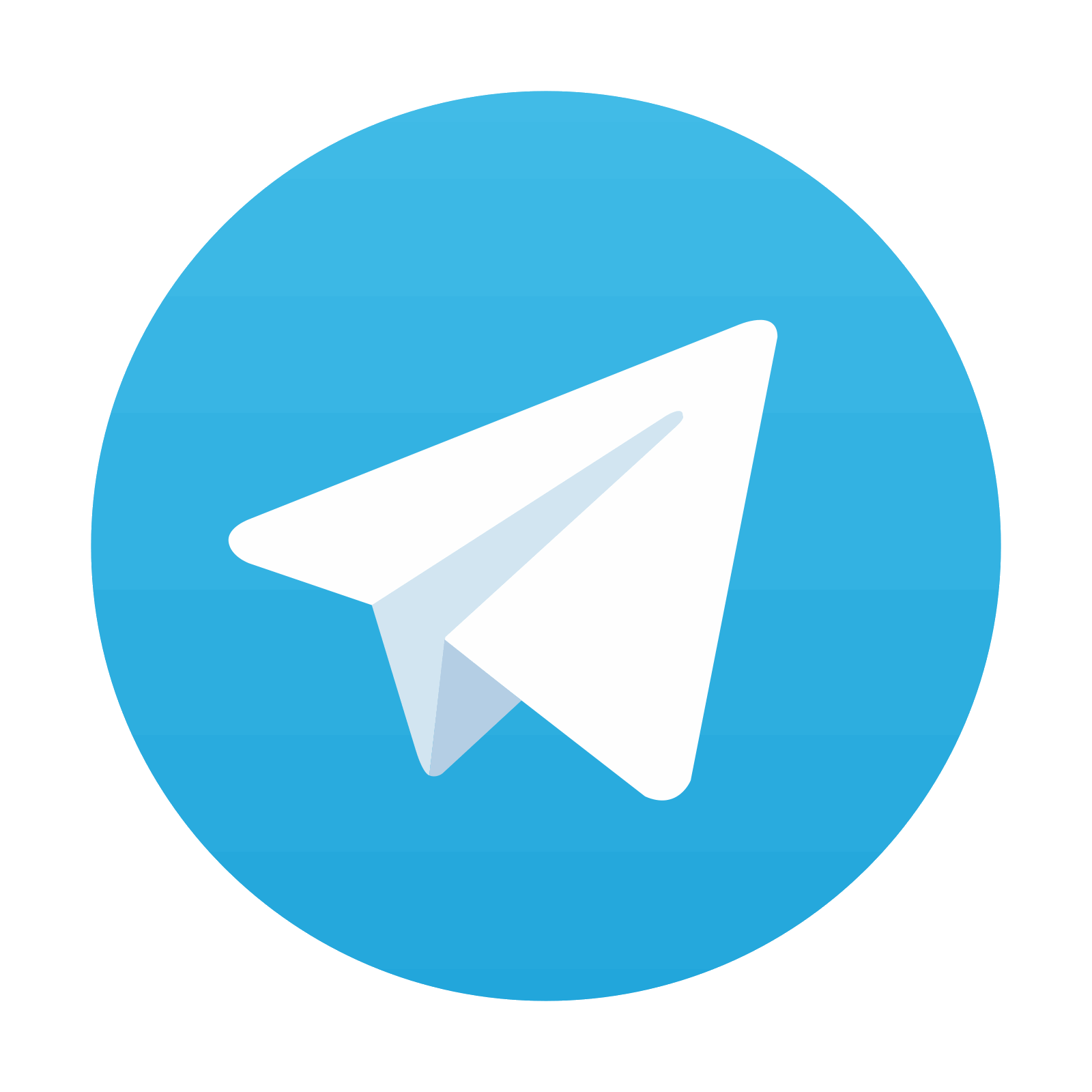
Stay updated, free articles. Join our Telegram channel
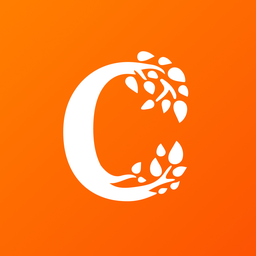
Full access? Get Clinical Tree
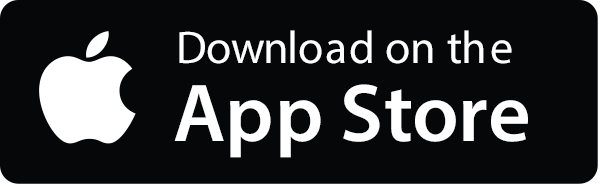
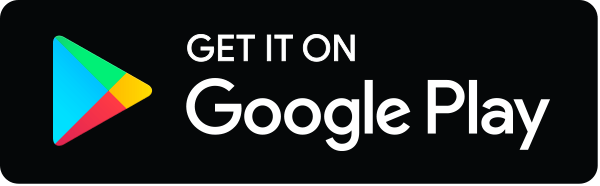