Biological Basis of Minimally Invasive Osteosynthesis
Saam Morshed
Carol A. Lin
Christian Krettek
Theodore Miclau III
INTRODUCTION
Prior to the era of aseptic technique and general anesthesia, the early treatment of fractures was almost entirely nonoperative. This avoided the risks of surgery; however, closed reduction and prolonged immobilization often led to joint stiffness, malunion, and impairment from disuse. The subsequent development of safer surgical practices led to increased operative management of fractures which initially called for anatomic reduction and rigid fixation to achieve primary cortical healing.1 While this allowed for early motion of the limb and perhaps the avoidance of debilitating permanent stiffness or “plaster disease,”2 the pursuit of precise anatomic reduction often resulted in significant operative trauma, increasing the risk of nonunion and infection.3,4
The emerging concept of “biologic fixation” aims to preserve the vascularity of the fracture and integrity of the fracture hematoma while achieving appropriate rigidity of the fracture repair construct to maximize healing potential.5, 6 and 7 This has led to the popularization of minimally invasive osteosynthesis (MIO) techniques that strive for the appropriate balance of soft tissue preservation and construct rigidity.8, 9, 10, 11, 12 and 13 This chapter provides an overview of the biology of fracture healing and the evidence supporting the concept of biologic fixation.
BIOLOGY OF FRACTURE REPAIR
Fracture repair involves a coordinated sequence of events involving four distinct phases: An initial inflammatory stage, soft callus stage, hard callus stage, and remodeling. The inflammatory stage involves hematoma formation allowing inflammatory cells to infiltrate and debride the fracture site and recruit cells necessary for bone repair. A soft callus stage composed of cartilage is formed next as these progenitor cells differentiate to form osteoblasts and chondrocytes. Chondrocytes then undergo maturation and the extracellular matrix is calcified. This is followed by removal of the calcified cartilage by osteoclasts and invasion by endothelial cells. The hard callus is formed, as bone is laid down behind the infiltrating vasculature. The newly formed bone is then remodeled until morphologically and mechanically similar to its preinjury state. Each of these phases of bone repair has been well studied in murine models14, 15, 16 and 17 and is explained in greater detail below.
At the time of fracture, torn periosteum, exposed bone marrow, and injured soft tissues bleed and create the fracture hematoma. This fracture hematoma contains inflammatory cells, including macrophages and platelets, that degranulate releasing inflammatory cytokines such as IL-1, IL-6,18 and TNFα19 and growth factors such as TGFβ, PDGF, and BMP.18 These molecules act on local cells in the marrow and periosteum to proliferate and differentiate.20 Within the first 7 to 10 days from injury, these mesenchymal cells aggregate and form condensations within the fracture hematoma and form cartilaginous tissue—the soft callus. Stem cells differentiate into chondrocytes or osteoblasts depending on the mechanical environment. Relative instability favors chondrocyte differentiation and endochondral ossification (Fig. 2.1), whereas stability favors osteoblast differentiation and intramembranous ossification (Fig. 2.2). Operative fracture fixation uses the entire spectrum from absolute rigid internal fixation to relative stability.
During this early period of fracture healing, the main extracellular components are type II collagen and proteoglycans. The proteoglycans inhibit mineralization of the mass until enough cartilage has been formed.21 These cells undergo proliferative and hypertrophic
phases identical to those that take place at the growth plate. Hypertrophic chondrocytes begin releasing vesicles containing calcium and proteolytic enzymes that release phosphate ions from the surrounding matrix and degrade proteoglycans. Through the precipitation of calcium and phosphate and the decreasing concentration of neighboring proteoglycans, the callus begins to mineralize.22 This process peaks at about 14 days and signals the beginning of vascular ingrowth into the fracture callus.23 The parallels between growth and fracture repair continue as the calcified cartilage is identical to the primary spongiosa at the growth plate. Between 2 and 3 weeks, chondroclasts remove the calcified cartilage as well as chondrocytes that have undergone apoptosis,24 and gradually osteoblasts convert the soft callus
to the hard callus by laying down woven bone that is identical to secondary spongiosa from the growth plate. This replacement process generally is completed by 3 to 4 weeks at which point the fracture is united. Osteoclasts then begin the remodeling process and the woven bone is converted to lamellar bone.
phases identical to those that take place at the growth plate. Hypertrophic chondrocytes begin releasing vesicles containing calcium and proteolytic enzymes that release phosphate ions from the surrounding matrix and degrade proteoglycans. Through the precipitation of calcium and phosphate and the decreasing concentration of neighboring proteoglycans, the callus begins to mineralize.22 This process peaks at about 14 days and signals the beginning of vascular ingrowth into the fracture callus.23 The parallels between growth and fracture repair continue as the calcified cartilage is identical to the primary spongiosa at the growth plate. Between 2 and 3 weeks, chondroclasts remove the calcified cartilage as well as chondrocytes that have undergone apoptosis,24 and gradually osteoblasts convert the soft callus
to the hard callus by laying down woven bone that is identical to secondary spongiosa from the growth plate. This replacement process generally is completed by 3 to 4 weeks at which point the fracture is united. Osteoclasts then begin the remodeling process and the woven bone is converted to lamellar bone.
The local soft tissues provide cellular and molecular elements that are critical to fracture repair: Skeletal progenitor cells derived from the periosteum and marrow differentiate into cartilage and bone; an extracellular matrix provides a scaffold for cells and storage space for cytokines and growth factors; and a blood supply provides the necessary cells, nutrition,
and molecules essential for healing. The periosteum is of primary importance as it contributes blood supply, undifferentiated mesenchymal cells as well as the osteoprogenitor cells that eventually become bone. On its own, the periosteum is capable of bridging gaps up to one-half the diameter of bone.25 Removal of the periosteum results in a weaker callus,26 and removal or compression of the periosteum overlying bone can cause bone necrosis.27, 28 and 29 The external soft tissues also contribute significantly to the blood supply that supports early callus formation and development.23,30,31 In poorly vascularized, hypoxic tissue, the differentiation and maturation of the chondrocytic tissue at the center of the callus fails and the callus cannot mature.32,33 In addition to local vascular supply, there is evidence in animal models suggesting that the nervous system may also play a role.34,35 The preservation of the soft tissue envelope and its vital functions is a fundamental component of biologic fracture fixation.
and molecules essential for healing. The periosteum is of primary importance as it contributes blood supply, undifferentiated mesenchymal cells as well as the osteoprogenitor cells that eventually become bone. On its own, the periosteum is capable of bridging gaps up to one-half the diameter of bone.25 Removal of the periosteum results in a weaker callus,26 and removal or compression of the periosteum overlying bone can cause bone necrosis.27, 28 and 29 The external soft tissues also contribute significantly to the blood supply that supports early callus formation and development.23,30,31 In poorly vascularized, hypoxic tissue, the differentiation and maturation of the chondrocytic tissue at the center of the callus fails and the callus cannot mature.32,33 In addition to local vascular supply, there is evidence in animal models suggesting that the nervous system may also play a role.34,35 The preservation of the soft tissue envelope and its vital functions is a fundamental component of biologic fracture fixation.
MECHANOBIOLOGY
In addition to fracture biology, the mechanical environment plays an important role in the cellular differentiation of the healing skeleton. Bone can successfully heal under both very rigid and relatively flexible conditions, and the exact relationship between local mechanical forces and the rate and method of fracture healing is still poorly understood. One prevailing concept as to how these factors interact is the interfragmentary strain theory proposed by Perren in 1979.36 Interfragmentary strain is defined as the ratio of the axial fracture displacement to the fracture gap width. The theory states that the method of cell differentiation during healing is determined by the amount of interfragmentary strain at the fracture site. In support of this hypothesis are observations that absence of instability results in minimal callus formation whereas small amounts of strain induce callus formation. Strain values of 2% are tolerated by lamellar bone tissues, while hard callus or woven bone can tolerate up to 10% strain. In contrast, granulation tissue will tolerate 100% strain before rupture.36,37 In order to obtain successful fracture union, the local environment must minimize the amount of interfragmentary strain. In the nonstabilized fracture, the increasing size and stiffness of callus reduce the amount of movement at the fracture ends, which allows for differentiation of cells to progressively stiffer tissue types. In experimental animal models, it has been shown that rigidly fixed fractures with very small gaps (i.e., areas of high strain) result in fracture ends that are resorbed prior to bony union.38,39
It should be noted, however, that Perren’s original hypothesis only considered axial deformation, whereas in vivo fractures are subjected to multiple directional forces when loaded. This has led to research evaluating the role of hydrostatic pressure,40 shear,41 and tensile stresses on fracture healing, as well as the timing and nature of loading. Cyclic, compressive axial loading across a gap can increase callus size and the rate of endochondral ossification.42, 43 and 44 Studies using distraction osteogenesis models have shown that static, tensile forces favor intramembranous ossification.45 In contrast, bending moments are known to favor cartilage formation and prevent ossification entirely.46,47 These studies are gradually clarifying the effects of the mechanical environment on fracture healing; however, many studies rely heavily on finite element analyses and assumed material properties.48
It is still unclear as to exactly how much motion and force will optimize fracture healing. Some assumptions appear to be consistent between a computational model and the more complex in vivo environment. Rigid fixation will depress callus formation and differentiation in the periosteum and soft tissues,25 whereas excessive motion and load may yield a hypertrophic nonunion.49,50 A small amount of cyclic motion and axial load in transverse fractures will accelerate healing,40,43,51 while shear forces in the same environment will result in nonunion.41,47 Although these relationships are still being actively investigated, it is clear that the surgeon must find the right balance of construct rigidity and soft tissue preservation in order to maximize bone healing potential.
THE CONCEPT OF BIOLOGIC FIXATION
As the interactions between fracture biology and mechanical stability are better understood, the central paradigm of direct reduction and absolute rigidity has given way to more soft
tissue sparing techniques. Where once the appearance of callus was thought to be a failure of fixation,52 its presence is now better appreciated in the healing of long bone fractures via flexible “internal splints.”53 Gerber et al.7 formalized the movement toward minimally invasive fracture fixation with the concept of “biologic fixation” in 1990, which “strives to obtain optimal rather than maximal stability with a minimum of soft tissue dissection.” While articular fragments still require anatomical reduction and rigid fixation, extra-articular fractures benefit from restoration of alignment and rotation with preservation of as much of the fracture hematoma and soft tissue envelope as possible.6,7,54
tissue sparing techniques. Where once the appearance of callus was thought to be a failure of fixation,52 its presence is now better appreciated in the healing of long bone fractures via flexible “internal splints.”53 Gerber et al.7 formalized the movement toward minimally invasive fracture fixation with the concept of “biologic fixation” in 1990, which “strives to obtain optimal rather than maximal stability with a minimum of soft tissue dissection.” While articular fragments still require anatomical reduction and rigid fixation, extra-articular fractures benefit from restoration of alignment and rotation with preservation of as much of the fracture hematoma and soft tissue envelope as possible.6,7,54
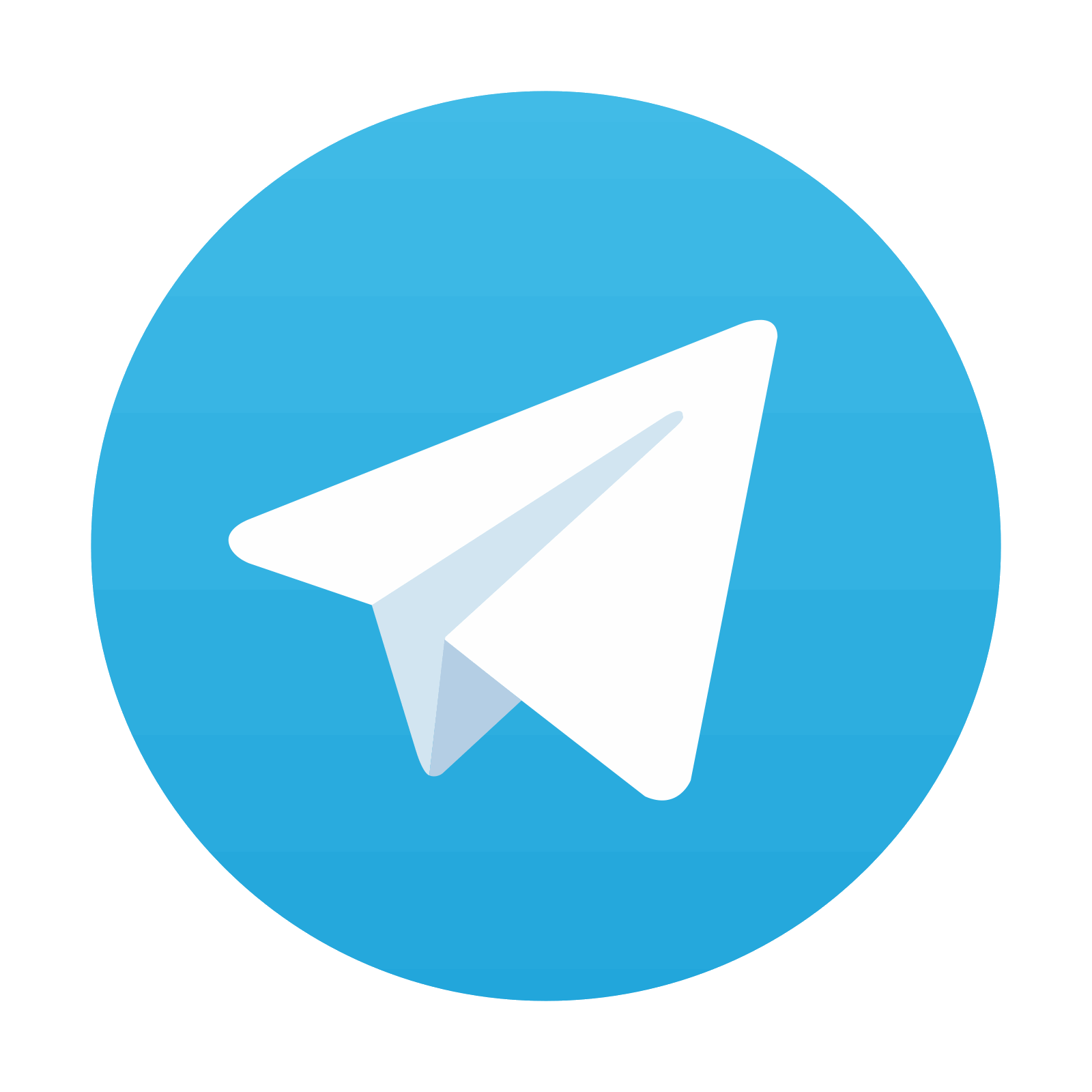
Stay updated, free articles. Join our Telegram channel
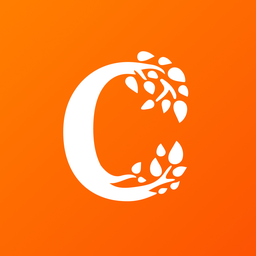
Full access? Get Clinical Tree
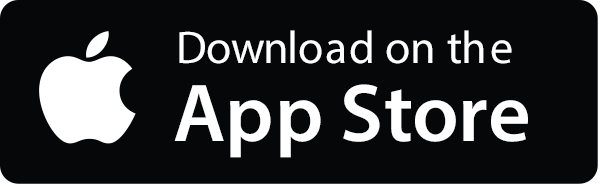
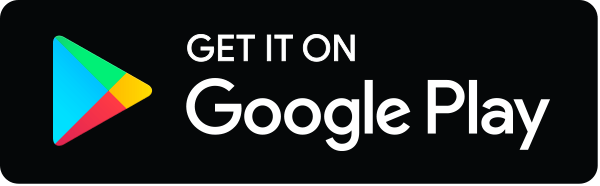